Diversity of Electron Transport Chains in Anaerobic Protists
Total Page:16
File Type:pdf, Size:1020Kb
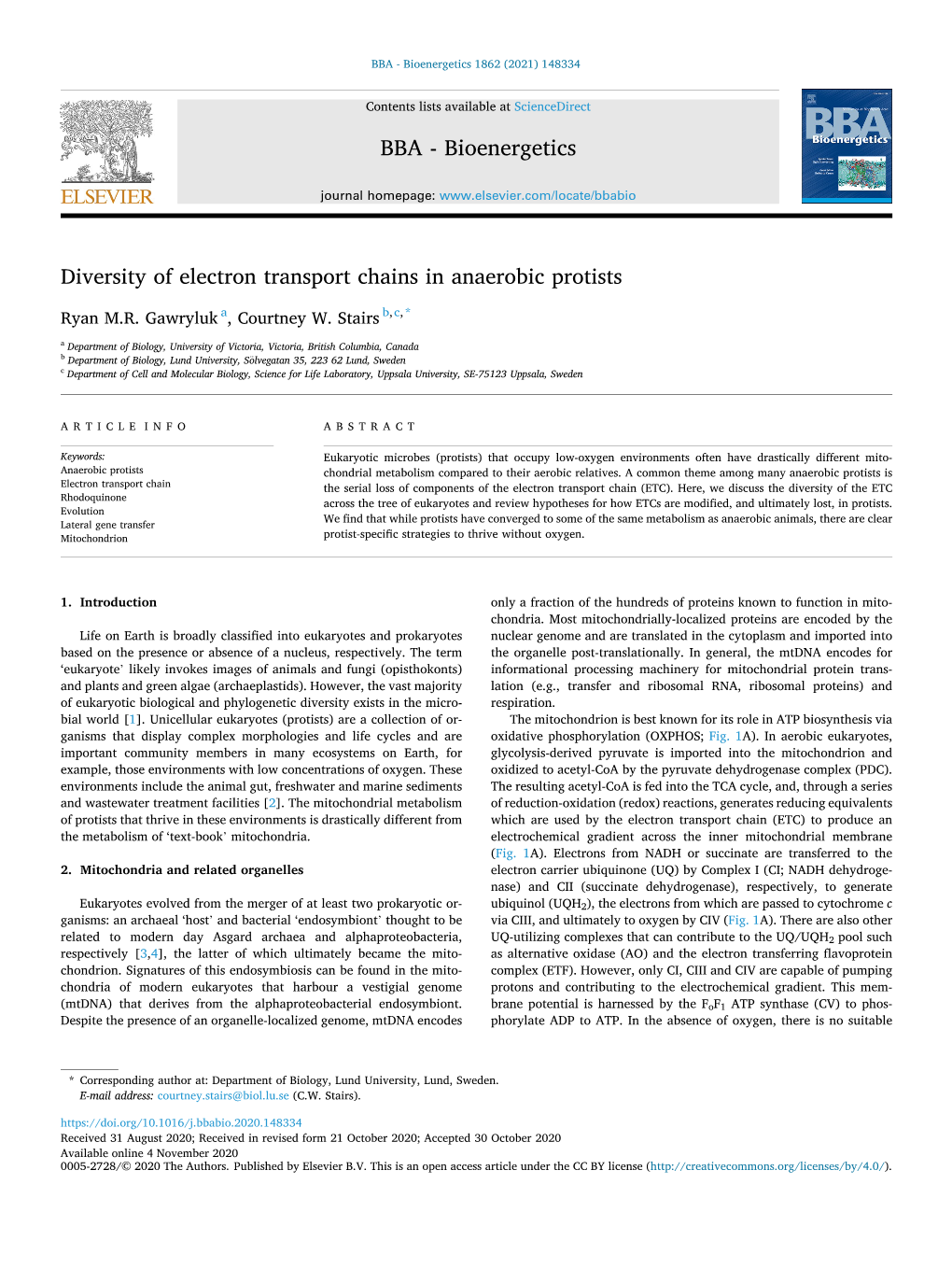
Load more
Recommended publications
-
Emerging Regulatory Roles of Mitochondrial Sirtuins on Pyruvate Dehydrogenase Complex and the Related Metabolic Diseases: Review
Biomedical Research and Therapy, 7(2):3645-3658 Open Access Full Text Article Review Emerging regulatory roles of mitochondrial sirtuins on pyruvate dehydrogenase complex and the related metabolic diseases: Review Abolfazl Nasiri1,2, Masoud Sadeghi3, Asad Vaisi-Raygani2,4, Sara Kiani3, Zahra Aghelan1,2, Reza Khodarahmi3,5,* ABSTRACT The pyruvate dehydrogenase complex (PDC) is a multi-enzyme complex of the mitochondria that provides a link between glycolysis and the Krebs cycle. PDC plays an essential role in producing Use your smartphone to scan this acetyl-CoA from glucose and the regulation of fuel consumption. In general, PDC enzyme is reg- QR code and download this article ulated in two different ways, end-product inhibition and posttranslational modifications (moreex- tensive phosphorylation and dephosphorylation subunit E1). Posttranslational modifications of this enzyme are regulated by various factors. Sirtuins are the class III of histone deacylatases that catalyze 1Students Research Committee, protein posttranslational modifications, including deacetylation, adenosine diphosphate ribosyla- Kermanshah University of Medical tion, and deacylation. Sirt3, Sirt4, and Sirt5 are mitochondrial sirtuins that control the posttrans- Sciences, Kermanshah, Iran lational modifications of mitochondrial protein. Considering the comprehensive role of sirtuins in post-translational modifications and regulation of metabolic processes, the aim of this review isto 2 Department of Clinical Biochemistry, explore the role of mitochondrial sirtuins in the regulation of the PDC. PDC deficiency is a com- School of Medicine, Kermanshah mon metabolic disorder that causes pyruvate to be converted to lactate and alanine rather than to University of Medical Sciences, Kermanshah, Iran acetyl-CoA. because this enzyme is in the gateway of complete oxidation, glucose products enter- ing the Krebs cycle and resulting in physiological and structural changes in the organs. -
Review Article Diversity of Eukaryotic Translational Initiation Factor Eif4e in Protists
Hindawi Publishing Corporation Comparative and Functional Genomics Volume 2012, Article ID 134839, 21 pages doi:10.1155/2012/134839 Review Article Diversity of Eukaryotic Translational Initiation Factor eIF4E in Protists Rosemary Jagus,1 Tsvetan R. Bachvaroff,2 Bhavesh Joshi,3 and Allen R. Place1 1 Institute of Marine and Environmental Technology, University of Maryland Center for Environmental Science, 701 E. Pratt Street, Baltimore, MD 21202, USA 2 Smithsonian Environmental Research Center, 647 Contees Wharf Road, Edgewater, MD 21037, USA 3 BridgePath Scientific, 4841 International Boulevard, Suite 105, Frederick, MD 21703, USA Correspondence should be addressed to Rosemary Jagus, [email protected] Received 26 January 2012; Accepted 9 April 2012 Academic Editor: Thomas Preiss Copyright © 2012 Rosemary Jagus et al. This is an open access article distributed under the Creative Commons Attribution License, which permits unrestricted use, distribution, and reproduction in any medium, provided the original work is properly cited. The greatest diversity of eukaryotic species is within the microbial eukaryotes, the protists, with plants and fungi/metazoa representing just two of the estimated seventy five lineages of eukaryotes. Protists are a diverse group characterized by unusual genome features and a wide range of genome sizes from 8.2 Mb in the apicomplexan parasite Babesia bovis to 112,000-220,050 Mb in the dinoflagellate Prorocentrum micans. Protists possess numerous cellular, molecular and biochemical traits not observed in “text-book” model organisms. These features challenge some of the concepts and assumptions about the regulation of gene expression in eukaryotes. Like multicellular eukaryotes, many protists encode multiple eIF4Es, but few functional studies have been undertaken except in parasitic species. -
Characterization of Aminoacyl-Trna Synthetases in Chromerids
Article Characterization of Aminoacyl-tRNA Synthetases in Chromerids Abdoallah Sharaf 1,2, Ansgar Gruber 1, Kateřina Jiroutová 1 and Miroslav Oborník 1,3,* 1 Institute of Parasitology, Biology Centre, Czech Academy of Sciences, 370 05 České Budějovice, Czech Republic 2 Genetics Department, Faculty of Agriculture, Ain Shams University, Cairo 11241, Egypt 3 Faculty of Science, University of South Bohemia, 370 05 České Budějovice, Czech Republic * Correspondence: [email protected] Received: 1 July 2019; Accepted: 28 July 2019; Published: 31 July 2019 Abstract: Aminoacyl-tRNA synthetases (AaRSs) are enzymes that catalyze the ligation of tRNAs to amino acids. There are AaRSs specific for each amino acid in the cell. Each cellular compartment in which translation takes place (the cytosol, mitochondria, and plastids in most cases), needs the full set of AaRSs; however, individual AaRSs can function in multiple compartments due to dual (or even multiple) targeting of nuclear- encoded proteins to various destinations in the cell. We searched the genomes of the chromerids, Chromera velia and Vitrella brassicaformis, for AaRS genes: 48 genes encoding AaRSs were identified in C. velia, while only 39 AaRS genes were found in V. brassicaformis. In the latter alga, ArgRS and GluRS were each encoded by a single gene occurring in a single copy; only PheRS was found in three genes, while the remaining AaRSs were encoded by two genes. In contrast, there were nine cases for which C. velia contained three genes of a given AaRS (45% of the AaRSs), all of them representing duplicated genes, except AsnRS and PheRS, which are more likely pseudoparalogs (acquired via horizontal or endosymbiotic gene transfer). -
Elevation of Cardiac Glycolysis Reduces Pyruvate Dehydrogenase but Increases Glucose Oxidation
University of Louisville ThinkIR: The University of Louisville's Institutional Repository Electronic Theses and Dissertations 5-2011 Elevation of cardiac glycolysis reduces pyruvate dehydrogenase but increases glucose oxidation. Qianwen Wang University of Louisville Follow this and additional works at: https://ir.library.louisville.edu/etd Recommended Citation Wang, Qianwen, "Elevation of cardiac glycolysis reduces pyruvate dehydrogenase but increases glucose oxidation." (2011). Electronic Theses and Dissertations. Paper 1519. https://doi.org/10.18297/etd/1519 This Doctoral Dissertation is brought to you for free and open access by ThinkIR: The University of Louisville's Institutional Repository. It has been accepted for inclusion in Electronic Theses and Dissertations by an authorized administrator of ThinkIR: The University of Louisville's Institutional Repository. This title appears here courtesy of the author, who has retained all other copyrights. For more information, please contact [email protected]. ELEVATION OF CARDIAC GLYCOLYSIS REDUCES PYRUVATE DEHYDROGENASE BUT INCREASES GLUCOSE OXIDATION By Qianwen Wang B.S., Guangdong Medical College of China, 1999 M.S., University of Louisville, 2006 A Dissertaion Submitted to the Faculty of the Graduate School of the University of Louisville In Partial Fulfillment of the Requirements for the Degree of Doctor of Philosophy Department of Physiology and Biophysics University of Louisville, School of Medicine Louisville, Kentucky May 2011 ELEVATION OF CARDIAC GLYCOLYSIS REDUCES PYRUVATE DEHYDROGENASE BUT INCREASES GLUCOSE OXIDATION By Qianwen Wang B.S., Guangdong Medical College of China, 1999 M.S., University of Louisville, 2006 A Dissertation Approved on March 28, 2011 by the following Dissertation Committee: Dissertation Director-Paul N. Epstein, Ph.D. William B. -
Cellular Respiration
Cellular Respiration Glucose is the preferred carbohydrate of cells. In solution, it can change from a linear chain to a ring. Energy is stored in the bonds of the carbohydrates. Breaking these bonds releases that energy. Crushing sugar crystals creates tiny electrical fields that give off invisible ultraviolet light. The wintergreen chemical (methyl salicylate) gets excited by these excited electrons and fluoresces in a visible blue wavelength. This phenomenon is called triboluminescence. Contents 1 Glycolysis 2 Fermentation 3 The Preparatory Reaction 4 Mitochondria 5 Aerobic Respiration 6 Metabolic Pool Glycolysis Glucose is the preferred carbohydrate of cells. Glycolysis (glyco – sugar; lysis – splitting) is a universal process of all cells that occurs in the cytosol whereby the glucose (a 6-carbon sugar) is split into two pyruvate (a 3-carbon molecule) molecules to generate ATP and reduced NADH. ATP (adenosine triphosphate) is the energy currency of the cell that stores chemical energy in 3 high energy phosphate bonds. NADH (reduced nicotinamide adenine dinucleotide) is a high energy electron carrier that acts as a coenzyme in reactions and as a rechargeable battery of sorts. The uncharged state that is not carrying high energy electrons is called NAD+. CC-BY-NC-SA | Jeremy Seto | New York City College of Technology | 1 Cellular Respiration Glycolysis is the splitting of glucose into 2 pyruvate molecules to generate 2 NADH and 2ATP molecules. ATP contains 3 high energy phosphates and acts as cellular energy currency. NADH is the reduced form of NAD+. The High energy electrons associated with the reduced form come with a H atom. -
CELLULAR RESPIRATION Applied Medical Chemistry II Course
CELLULAR RESPIRATION Applied Medical Chemistry II Course Aim of the lecture This lecture discuss the steps of cellular respiration and its regulation Maye M. Ragab Super Visor / Dr. Neveen Introduction individual enzymic reactions were analyzed in an effort to explain the mechanisms of catalysis. However, in cells, these reactions rarely occur in isolation, but rather are organized into multistep sequences called pathways). In a pathway, the product of one reaction serves as the substrate of the subsequent reaction. Different pathways can also intersect, forming an integrated and purposeful network of chemical reactions. These are collectively called metabolism, which is the sum of all the chemical changes occurring in a cell, a tissue, or the body. Most pathways can be classified as either catabolic (degradative) or anabolic (synthetic). Anabolic pathways • form complex end products from simple precursors, for example, the synthesis of the polysaccharide, glycogen, from glucose. • Anabolic reactions require energy (are endergonic), which is generally provided by the breakdown of ATP to (ADP) and inorganic phosphate (Pi). • Anabolic reactions often involve chemical reductions in which the reducing power is most frequently provided by the electron donor NADPH . Catabolic reactions • break down complex molecules, such as proteins, polysaccharides, and lipids, to a few simple molecules, for example, CO2, NH3 (ammonia), and water. • Catabolic reactions serve to capture chemical energy in the form of (ATP) from the degradation of energy-rich fuel molecules. Energy generation by degradation of complex molecules occurs in three stages as in the following figure : [Note: Catabolic pathways are typically oxidative, and require coenzymes such as NAD+.] [Note: Pathways that regenerate a component are called cycles.] 1 2 Overview Of Glycolysis : • The glycolytic pathway is employed by all tissues for the breakdown of glucose to provide energy (in the form of ATP) and intermediates for other metabolic pathways. -
Downloaded from the Uni- [76] and Kept Only the Best Match with the Delta-Filter Protkb [85] Databank (9/2014) Were Aligned to the Gen- Command
Farhat et al. BMC Biology (2021) 19:1 https://doi.org/10.1186/s12915-020-00927-9 RESEARCH ARTICLE Open Access Rapid protein evolution, organellar reductions, and invasive intronic elements in the marine aerobic parasite dinoflagellate Amoebophrya spp Sarah Farhat1,2† , Phuong Le,3,4† , Ehsan Kayal5† , Benjamin Noel1† , Estelle Bigeard6, Erwan Corre5 , Florian Maumus7, Isabelle Florent8 , Adriana Alberti1, Jean-Marc Aury1, Tristan Barbeyron9, Ruibo Cai6, Corinne Da Silva1, Benjamin Istace1, Karine Labadie1, Dominique Marie6, Jonathan Mercier1, Tsinda Rukwavu1, Jeremy Szymczak5,6, Thierry Tonon10 , Catharina Alves-de-Souza11, Pierre Rouzé3,4, Yves Van de Peer3,4,12, Patrick Wincker1, Stephane Rombauts3,4, Betina M. Porcel1* and Laure Guillou6* Abstract Background: Dinoflagellates are aquatic protists particularly widespread in the oceans worldwide. Some are responsible for toxic blooms while others live in symbiotic relationships, either as mutualistic symbionts in corals or as parasites infecting other protists and animals. Dinoflagellates harbor atypically large genomes (~ 3 to 250 Gb), with gene organization and gene expression patterns very different from closely related apicomplexan parasites. Here we sequenced and analyzed the genomes of two early-diverging and co-occurring parasitic dinoflagellate Amoebophrya strains, to shed light on the emergence of such atypical genomic features, dinoflagellate evolution, and host specialization. Results: We sequenced, assembled, and annotated high-quality genomes for two Amoebophrya strains (A25 and A120), using a combination of Illumina paired-end short-read and Oxford Nanopore Technology (ONT) MinION long-read sequencing approaches. We found a small number of transposable elements, along with short introns and intergenic regions, and a limited number of gene families, together contribute to the compactness of the Amoebophrya genomes, a feature potentially linked with parasitism. -
The PEP–Pyruvate–Oxaloacetate Node As the Switch Point for Carbon flux Distribution in Bacteria
View metadata, citation and similar papers at core.ac.uk brought to you by CORE provided by RERO DOC Digital Library FEMS Microbiology Reviews 29 (2005) 765–794 www.fems-microbiology.org The PEP–pyruvate–oxaloacetate node as the switch point for carbon flux distribution in bacteria Uwe Sauer a, Bernhard J. Eikmanns b,* a Institute of Biotechnology, ETH Zu¨rich, 8093 Zu¨rich, Switzerland b Department of Microbiology and Biotechnology, University of Ulm, 89069 Ulm, Germany Received 18 August 2004; received in revised form 27 October 2004; accepted 1 November 2004 First published online 28 November 2004 We dedicate this paper to Rudolf K. Thauer, Director of the Max-Planck-Institute for Terrestrial Microbiology in Marburg, Germany, on the occasion of his 65th birthday Abstract In many organisms, metabolite interconversion at the phosphoenolpyruvate (PEP)–pyruvate–oxaloacetate node involves a struc- turally entangled set of reactions that interconnects the major pathways of carbon metabolism and thus, is responsible for the dis- tribution of the carbon flux among catabolism, anabolism and energy supply of the cell. While sugar catabolism proceeds mainly via oxidative or non-oxidative decarboxylation of pyruvate to acetyl-CoA, anaplerosis and the initial steps of gluconeogenesis are accomplished by C3- (PEP- and/or pyruvate-) carboxylation and C4- (oxaloacetate- and/or malate-) decarboxylation, respectively. In contrast to the relatively uniform central metabolic pathways in bacteria, the set of enzymes at the PEP–pyruvate–oxaloacetate node represents a surprising diversity of reactions. Variable combinations are used in different bacteria and the question of the sig- nificance of all these reactions for growth and for biotechnological fermentation processes arises. -
In Vivo Assessment of Pyruvate Dehydrogenase Flux in the Heart Using Hyperpolarized Carbon-13 Magnetic Resonance
In vivo assessment of pyruvate dehydrogenase flux in the heart using hyperpolarized carbon-13 magnetic resonance Marie A. Schroeder, Lowri E. Cochlin, Lisa C. Heather, Kieran Clarke, George K. Radda, and Damian J. Tyler* Cardiac Metabolism Research Group, Department of Physiology, Anatomy, and Genetics, University of Oxford, Oxford OX1 3PT, United Kingdom Communicated by Robert G. Shulman, Yale University, New Haven, CT, June 19, 2008 (received for review January 15, 2008) The advent of hyperpolarized 13C magnetic resonance (MR) has substrate composition can alter the phosphorylation state and provided new potential for the real-time visualization of in vivo thus activity of the PDH enzyme complex (7). Elevated insulin metabolic processes. The aim of this work was to use hyperpolar- concentrations are thought to stimulate PDP acutely, thereby ized [1-13C]pyruvate as a metabolic tracer to assess noninvasively dephosphorylating and activating PDH (9). The relative activi- the flux through the mitochondrial enzyme complex pyruvate ties of PDK and PDP determine the proportion of PDH that is dehydrogenase (PDH) in the rat heart, by measuring the produc- in the active dephosphorylated form. ؊ 13 tion of bicarbonate (H CO3 ), a byproduct of the PDH-catalyzed The link between PDH regulation and disease has made the conversion of [1-13C]pyruvate to acetyl-CoA. By noninvasively assessment of substrate selection and PDH activity important ؊ 13 observing a 74% decrease in H CO3 production in fasted rats benchmarks in the study of heart disease. To date, measurement compared with fed controls, we have demonstrated that hyper- of cardiac PDH activity has been performed with invasive 13 polarized C MR is sensitive to physiological perturbations in PDH biochemical assays, whereas substrate selection has been mon- 13 flux. -
Pyruvate Ferredoxin Oxidoreductase from the Hyperthermophilic
Proc. Natl. Acad. Sci. USA Vol. 94, pp. 9608–9613, September 1997 Biochemistry Pyruvate ferredoxin oxidoreductase from the hyperthermophilic archaeon, Pyrococcus furiosus, functions as a CoA-dependent pyruvate decarboxylase (archaeayaldehydeydecarboxylationy2-keto acidythiamine pyrophosphate) KESEN MA*, ANDREA HUTCHINS*, SHI-JEAN S. SUNG†, AND MICHAEL W. W. ADAMS*‡ *Center for Metalloenzyme Studies, Department of Biochemistry and Molecular Biology, University of Georgia, Athens, GA 30602; and †Institute of Tree Root Biology, U.S. Department of Agriculture–Forest Service, Athens, GA 30602 Communicated by Gregory A. Petsko, Brandeis University, Waltham, MA, June 17, 1997 (received for review June 1, 1996) ABSTRACT Pyruvate ferredoxin oxidoreductase (POR) hyperthermophilic archaea and these are involved in peptide has been previously purified from the hyperthermophilic fermentation. They use 2-ketoglutarate (KGOR) (11), in- archaeon, Pyrococcus furiosus, an organism that grows opti- dolepyruvate (IOR) (12), and 2-ketoisovalerate (VOR) (13) as mally at 100°C by fermenting carbohydrates and peptides. The substrates, and function to oxidatively decarboxylate the 2- enzyme contains thiamine pyrophosphate and catalyzes the keto acids generated by the transamination of glutamate, oxidative decarboxylation of pyruvate to acetyl-CoA and CO2 aromatic amino acids, and branched chain amino acids, re- and reduces P. furiosus ferredoxin. Here we show that this spectively, to the corresponding CoA derivative (13). enzyme also catalyzes the formation of acetaldehyde from The growth of hyperthermophilic archaea such as P. furiosus pyruvate in a CoA-dependent reaction. Desulfocoenzyme A is also unusual in that it is dependent upon tungsten (14), a substituted for CoA showing that the cofactor plays a struc- metal seldom used in biological systems (15). -
The Citric Acid Cycle Also Known As Tricarboxylic Acid Cycle TCA Krebs Cycle
ATP-ADP Translocase The flows of ATP and ADP are coupled (ADP enters only if ATP exits Highly abundant (14% of IMM proteins) Contains a single nucleotide-binding site (alternates) Similar affinity to ATP and ADP Endergonic (25% of ETC) Inhibition leads to subsequent inhibition of cellular respiration For every 2 electrons passing: 4H+ (complex I); 0H+ (complex II); 4H+ (complex III), 2H+ (complex IV) The citric acid cycle also known as Tricarboxylic acid cycle TCA Krebs cycle Lippincott chapter 9 Krebs cycle is the final pathway where the oxidative metabolism of carbohydrates, amino acids, and fatty acids convert their carbon skeletons to CO2. Most of the body's catabolic pathways converge on the TCA cycle (C3) From glycolysis to TCA Cycle Oxidative decarboxylation of pyruvate (C2) In the mitochondrial matrix, pyruvate undergoes oxidative decarboxylation to form acetyl CoA. TCA Cycle Summary From Pyruvate to Acetyl Co-A Pyruvate Pyruvate dehydrogenase complex (a multienzyme Dehydrogenase complex) The Formation of Acetyl CoA from Pyruvate • Irreversible reaction • Pyruvate Dehydrogenase complex: large, complex of three kinds of enzymes • Five coenzymes: – thiamine pyrophosphate (TPP), – lipoic acid, – FAD – CoA – NAD+. • Two additional enzymes regulate its activity Pyruvate Decarboxylation Steps Step 1: Decarboxylation Step 2: Oxidation Lipoic acid is attached to the enzyme by amide bond lipoamide Can be oxidized or reduced Step 3: Transfer of the resultant acetyl group to CoA Oxidation Conversion of pyruvate into acetyl CoA consists of three steps: decarboxylation, oxidation, and transfer of the resultant acetyl group to CoA. The mechanism of the pyruvate dehydrogenase reaction is more complex than is suggested by its relatively simple stoichiometry. -
CURRICULUM VITAE Saddef Haq, Ph.D. Program in Toxicology University of Maryland Baltimore School of Medicine
Beyond the Dinoflagellate Transcriptome: Validation of Protein Production via Biochemical Analysis and Mass Spectrometry Item Type dissertation Authors Haq, Saddef Publication Date 2018 Abstract Dinoflagellates are members of the Alveolata (meaning “with cavities”), a monophyletic group of single cell protists which includes apicomplexans and ciliates that exhibit a diverse mode of nutrition, ranging from predation to photo autotrophy to int... Keywords bioluminescence; dinoflagellates; toxin synthesis; Acetyl-CoA Carboxylase; Dinoflagellida; Polyketide Synthases; Proteomics Download date 27/09/2021 16:23:13 Link to Item http://hdl.handle.net/10713/8970 CURRICULUM VITAE Saddef Haq, Ph.D. Program in Toxicology University of Maryland Baltimore School of Medicine Institute of Marine and Environmental Technology 701 E. Pratt Street Baltimore, Maryland 21202 Email: [email protected] EDUCATION 2013-2018 University of Maryland, Baltimore, MD Program in Toxicology Doctor of Philosophy 2006-2008 Rutgers University, New Brunswick, NJ Bachelor of Science in Biochemistry 2003-2006 Union County College, Cranford, NJ Associates of Science in Biology EMPLOYMENT HISTORY July 2013-Present Graduate Research Assistant Graduate Program in Life Sciences, Program in Toxicology University of Maryland, Baltimore, Baltimore, MD Lab of Dr. Allen R. Place at the Institute of Marine and Environmental Technology, Baltimore, MD. Sep 2010-May 2013 Senior Research Technician Center for Infection and Immunity Columbia University, School of Public Health New York, NY