Simanonokm1218.Pdf (2.150Mb)
Total Page:16
File Type:pdf, Size:1020Kb
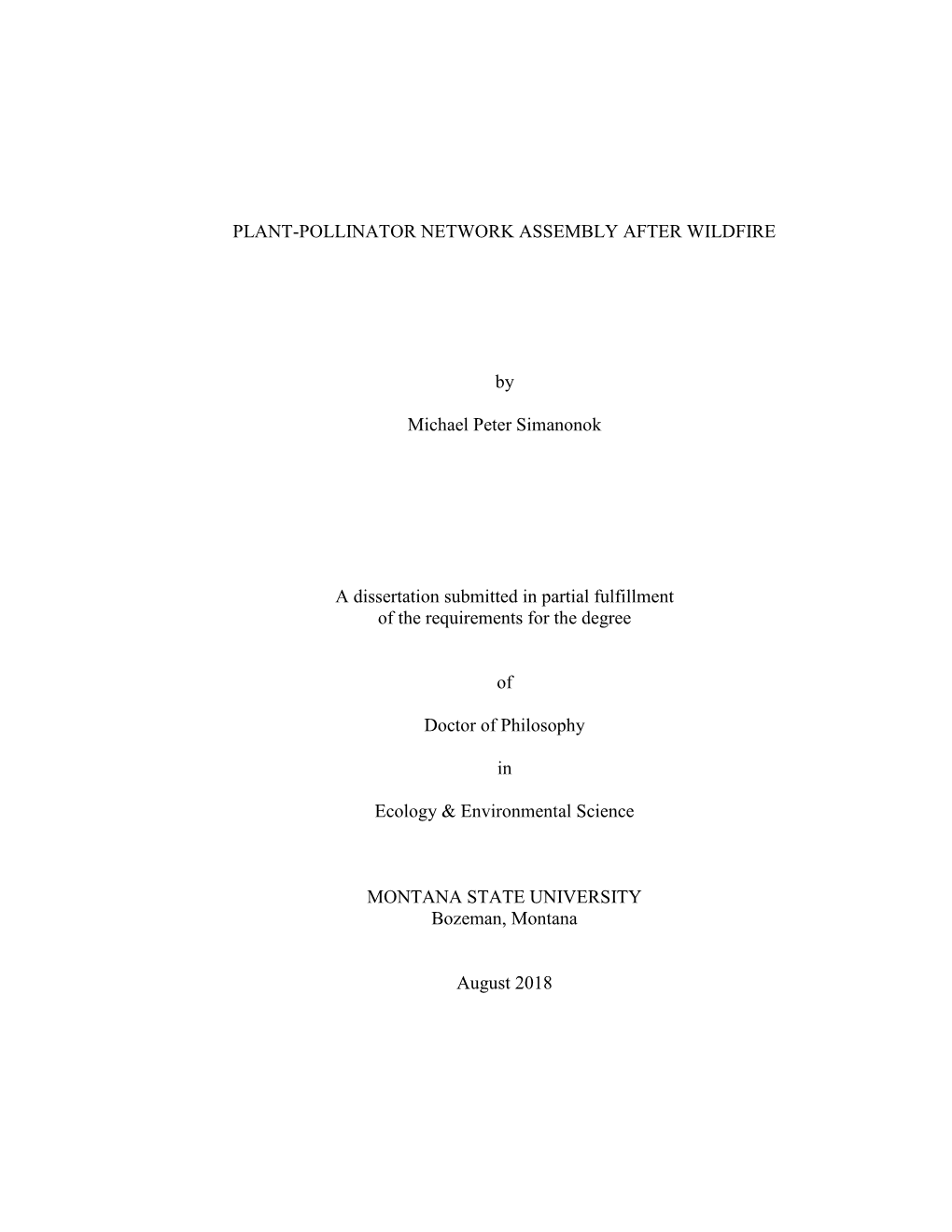
Load more
Recommended publications
-
Review of the Diet and Micro-Habitat Values for Wildlife and the Agronomic Potential of Selected Grassland Plant Species
Report Number 697 Review of the diet and micro-habitat values for wildlifeand the agronomic potential of selected grassland plant species English Nature Research Reports working today for nature tomorrow English Nature Research Reports Number 697 Review of the diet and micro-habitat values for wildlife and the agronomic potential of selected grassland plant species S.R. Mortimer, R. Kessock-Philip, S.G. Potts, A.J. Ramsay, S.P.M. Roberts & B.A. Woodcock Centre for Agri-Environmental Research University of Reading, PO Box 237, Earley Gate, Reading RG6 6AR A. Hopkins, A. Gundrey, R. Dunn & J. Tallowin Institute for Grassland and Environmental Research North Wyke Research Station, Okehampton, Devon EX20 2SB J. Vickery & S. Gough British Trust for Ornithology The Nunnery, Thetford, Norfolk IP24 2PU You may reproduce as many additional copies of this report as you like for non-commercial purposes, provided such copies stipulate that copyright remains with English Nature, Northminster House, Peterborough PE1 1UA. However, if you wish to use all or part of this report for commercial purposes, including publishing, you will need to apply for a licence by contacting the Enquiry Service at the above address. Please note this report may also contain third party copyright material. ISSN 0967-876X © Copyright English Nature 2006 Project officer Heather Robertson, Terrestrial Wildlife Team [email protected] Contractor(s) (where appropriate) S.R. Mortimer, R. Kessock-Philip, S.G. Potts, A.J. Ramsay, S.P.M. Roberts & B.A. Woodcock Centre for Agri-Environmental Research, University of Reading, PO Box 237, Earley Gate, Reading RG6 6AR A. -
Notes on Megachile Centuncularis
Utah State University DigitalCommons@USU Ga Bee Lab 1-1-1874 Notes on Megachile centuncularis Thos. G. Gentry Follow this and additional works at: https://digitalcommons.usu.edu/bee_lab_ga Part of the Entomology Commons Recommended Citation Gentry, Thos. G., "Notes on Megachile centuncularis" (1874). Ga. Paper 128. https://digitalcommons.usu.edu/bee_lab_ga/128 This Article is brought to you for free and open access by the Bee Lab at DigitalCommons@USU. It has been accepted for inclusion in Ga by an authorized administrator of DigitalCommons@USU. For more information, please contact [email protected]. 170 'fHE CANADIAN ENTOMOLOGIST. 171 paler than the wings, but I can at once of dijfi11is. But the terminal segments in dijfinis are not " ferru-• Al. ex. -.¡\ inch. ginous" any more than in tmiformis, and so Kirby may have had a boreal The larva is white, without maculae, but with the anterior rnargin oí specieswe do not yet know before him. From his description there is. nomore correspondence with 1mifarmis than with tl1ysbe; rather <loes his. the first segrnent b;own. _ description agree with f11scica11disas to the abdomen terminally. A. l1ydranga:ella. N. sp. ' Cressonia juglandis, p. iv. To this species must be cited Sm. -pallens- The mine and larva only of this species is known, and o( Mr. Strecker, whose figure represents a ?ale ~ specimen of C. succeeded in rearing the imago. The mine, larva and case resernble those j,tgla11dis,without the median shade on thc forewings. Belfrage has sent of A. 11iticordifalie/la, but are perhaps a little srnaller. It mines the leavcs C.j11gla11dis from Texas. -
Flower Preferences and Pollen Transport Networks for Cavity‐Nesting Solitary Bees: Implications for the Design of Agri‐Envir
Received: 14 February 2018 | Revised: 22 April 2018 | Accepted: 23 April 2018 DOI: 10.1002/ece3.4234 ORIGINAL RESEARCH Flower preferences and pollen transport networks for cavity- nesting solitary bees: Implications for the design of agri- environment schemes Catherine E. A. Gresty1 | Elizabeth Clare2 | Dion S. Devey3 | Robyn S. Cowan3 | Laszlo Csiba3 | Panagiota Malakasi3 | Owen T. Lewis1 | Katherine J. Willis1,3 1Department of Zoology, University of Oxford, Oxford, UK Abstract 2School of Biological and Chemical Floral foraging resources are valuable for pollinator conservation on farmland, and Sciences, Queen Mary University of London, their provision is encouraged by agri- environment schemes in many countries. Across London, UK Europe, wildflower seed mixtures are widely sown on farmland to encourage pollina- 3Royal Botanic Gardens, Kew, Richmond, UK tors, but the extent to which key pollinator groups such as solitary bees exploit and Correspondence benefit from these resources is unclear. We used high- throughput sequencing of 164 Catherine E. A. Gresty, Department of Zoology, New Radcliffe House, Radcliffe pollen samples extracted from the brood cells of six common cavity- nesting solitary Observatory Quarter, 6GG, Woodstock Rd, bee species (Osmia bicornis, Osmia caerulescens, Megachile versicolor, Megachile Oxford OX2, UK. Email: [email protected] ligniseca, Megachile centuncularis and Hylaeus confusus) which are widely distributed across the UK and Europe. We documented their pollen use across 19 farms in south- ern England, UK, revealing their forage plants and examining the structure of their pollen transport networks. Of the 32 plant species included currently in sown wild- flower mixes, 15 were recorded as present within close foraging range of the bees on the study farms, but only Ranunculus acris L. -
Leafcutting Bees, Megachilidae (Insecta: Hymenoptera: Megachilidae: Megachilinae)1 David Serrano2
EENY-342 Leafcutting Bees, Megachilidae (Insecta: Hymenoptera: Megachilidae: Megachilinae)1 David Serrano2 Introduction Distribution Leafcutting bees are important native pollinators of North Leafcutting bees are found throughout the world and America. They use cut leaves to construct nests in cavities are common in North America. In Florida there are ap- (mostly in rotting wood). They create multiple cells in the proximately 63 species (plus five subspecies) within seven nest, each with a single larva and pollen for the larva to eat. genera of leafcutter bees: Ashmeadiella, Heriades, Hoplitis, Leafcutting bees are important pollinators of wildflowers, Coelioxys, Lithurgus, Megachile, and Osmia. fruits, vegetables and other crops. Some leafcutting bees, Osmia spp., are even used as commercial pollinators (like Description honey bees) in crops such as alfalfa and blueberries. Most leafcutting bees are moderately sized (around the size of a honey bee, ranging from 5 mm to 24 mm), stout-bod- ied, black bees. The females, except the parasitic Coelioxys, carry pollen on hairs on the underside of the abdomen rather than on the hind legs like other bees. When a bee is carrying pollen, the underside of the abdomen appears light yellow to deep gold in color. Biology Leafcutting bees, as their name implies, use 0.25 to 0.5 inch circular pieces of leaves they neatly cut from plants to construct nests. They construct cigar-like nests that contain several cells. Each cell contains a ball or loaf of stored pollen and a single egg. Therefore, each cell will produce a Figure 1. A leafcutting bee, Megachile sp. single bee. -
Tracking Plant Phenology and Pollinator Diversity Across Alaskan National Parks a Pilot Study
National Park Service U.S. Department of the Interior Natural Resource Stewardship and Science Tracking Plant Phenology and Pollinator Diversity Across Alaskan National Parks A Pilot Study Natural Resource Report NPS/AKRO/NRR—2021/2291 ON THE COVER Clockwise from top left: A. Mocorro Powell collecting pollinators in Denali NPP; long-horned beetle on common yarrow; K. Fuentes scoring phenophases on common yarrow in Klondike Gold Rush NHP; bumble bee on fireweed NPS/Jessica Rykken Tracking Plant Phenology and Pollinator Diversity Across Alaskan National Parks A Pilot Study Natural Resource Report NPS/AKRO/NRR—2021/2291 Jessica J. Rykken National Park Service Denali National Park and Preserve PO Box 9 Denali Park, AK 99755 August 2021 U.S. Department of the Interior National Park Service Natural Resource Stewardship and Science Fort Collins, Colorado The National Park Service, Natural Resource Stewardship and Science office in Fort Collins, Colorado, publishes a range of reports that address natural resource topics. These reports are of interest and applicability to a broad audience in the National Park Service and others in natural resource management, including scientists, conservation and environmental constituencies, and the public. The Natural Resource Report Series is used to disseminate comprehensive information and analysis about natural resources and related topics concerning lands managed by the National Park Service. The series supports the advancement of science, informed decision-making, and the achievement of the National Park Service mission. The series also provides a forum for presenting more lengthy results that may not be accepted by publications with page limitations. All manuscripts in the series receive the appropriate level of peer review to ensure that the information is scientifically credible, technically accurate, appropriately written for the intended audience, and designed and published in a professional manner. -
Unique Bee Communities Within Vacant Lots and Urban Farms Result from Variation in Surrounding Urbanization Intensity
sustainability Article Unique Bee Communities within Vacant Lots and Urban Farms Result from Variation in Surrounding Urbanization Intensity Frances S. Sivakoff ID , Scott P. Prajzner and Mary M. Gardiner * ID Department of Entomology, The Ohio State University, 2021 Coffey Road, Columbus, OH 43210, USA; [email protected] (F.S.S.); [email protected] (S.P.P.) * Correspondence: [email protected]; Tel.: +1-330-601-6628 Received: 1 May 2018; Accepted: 5 June 2018; Published: 8 June 2018 Abstract: We investigated the relative importance of vacant lot and urban farm habitat features and their surrounding landscape context on bee community richness, abundance, composition, and resource use patterns. Three years of pan trap collections from 16 sites yielded a rich assemblage of bees from vacant lots and urban farms, with 98 species documented. We collected a greater bee abundance from vacant lots, and the two forms of greenspace supported significantly different bee communities. Plant–pollinator networks constructed from floral visitation observations revealed that, while the average number of bees utilizing available resources, niche breadth, and niche overlap were similar, the composition of floral resources and common foragers varied by habitat type. Finally, we found that the proportion of impervious surface and number of greenspace patches in the surrounding landscape strongly influenced bee assemblages. At a local scale (100 m radius), patch isolation appeared to limit colonization of vacant lots and urban farms. However, at a larger landscape scale (1000 m radius), increasing urbanization resulted in a greater concentration of bees utilizing vacant lots and urban farms, illustrating that maintaining greenspaces provides important habitat, even within highly developed landscapes. -
Bee Species Checklist of the San Francisco Peaks, Arizona
Biodiversity Data Journal 8: e49285 doi: 10.3897/BDJ.8.e49285 Taxonomic Paper Bee species checklist of the San Francisco Peaks, Arizona Lindsie M McCabe‡, Paige R Chesshire‡§, David R Smith , Atticus Wolf‡, Jason Gibbs |, Terry L Griswold¶, Karen W Wright#‡, Neil S Cobb ‡ Department of Biological Sciences, Northern Arizona University, Flagstaff, United States of America § U.S. Fish and Wildlife Service, Southwest Forest Science Complex, Flagstaff, United States of America | Department of Entomology, University of Manitoba, Winnipeg, Canada ¶ USDA-ARS, Pollinating Insects Research Unit, Logan, United States of America # Department of Entomology, Texas A&M, College Station, United States of America Corresponding author: Lindsie M McCabe ([email protected]) Academic editor: Dominique Zimmermann Received: 11 Dec 2019 | Accepted: 25 Mar 2020 | Published: 02 Apr 2020 Citation: McCabe LM, Chesshire PR, Smith DR, Wolf A, Gibbs J, Griswold TL, Wright KW, Cobb NS (2020) Bee species checklist of the San Francisco Peaks, Arizona. Biodiversity Data Journal 8: e49285. https://doi.org/10.3897/BDJ.8.e49285 Abstract Background Here we present a checklist of the bee species found on the C. Hart Merriam elevation gradient along the San Francisco Peaks in northern Arizona. Elevational gradients can serve as natural proxies for climate change, replacing time with space as they span multiple vegetation zones over a short geographic distance. Describing the distribution of bee species along this elevation gradient will help predict how bee communities might respond to changing climate. To address this, we initiated an inventory associated with ecological studies on pollinators that documented bees on the San Francisco Peaks. -
Bees (Hymenoptera: Apoidea, Apiformes) of the Kujawy Lakeland (Central Poland)
J. Banaszak and A. Sobieraj-Betlińska FRAGMENTA FAUNISTICA 59 (1): 7–27, 2016 PL ISSN 0015-9301 © MUSEUM AND INSTITUTE OF ZOOLOGY PAS DOI 10.3161/00159301FF2016.59.1.007 Bees (Hymenoptera: Apoidea, Apiformes) of the Kujawy Lakeland (central Poland) Józef BANASZAK and Anna SOBIERAJ-BETLIŃSKA Department of Ecology, Institute of Environmental Biology, Kazimierz Wielki University,12 Ossolińskich Av., 85-093 Bydgoszcz, Poland; e-mail: [email protected], [email protected] Abstract: Bee diversity was studied in 14 habitats in 7 localities in the Kujawy Lakeland (Pojezierze Kujawskie) in central Poland. Additionally, we investigated the species diversity and phenology of bumblebees on red clover (Trifolium pratense). In total, 146 bee species were recorded in the study area, accounting for 30.7% of bee species reported from Poland so far and 46.2% of bee species known from the Wielkopolska-Kujawy Lowland (Nizina Wielkopolsko-Kujawska). These include 14 red-listed species. Key words: wild bees, Pojezierze Kujawskie, Nadgoplański Park Tysiąclecia, dominance structure, species occurrence INTRODUCTION Kujawy Lakeland (Pojezierze Kujawskie) is located in central Poland and constitutes the south-eastern part of the Wielkopolska Lakeland (Pojezierze Wielkopolskie). We chose this study area because its bee fauna was not investigated before. Earlier publications included a lot of information on bees in the Wielkopolska-Kujawy Lowland (Nizina Wielkopolsko- Kujawska) (Alfken 1909, 1912, Torka 1913, 1933, Szulczewski 1948, Banaszak 1982, 1983, 1987, Pawlikowski 1989a, 1989b, 1992a, 1992b, 1993), but excluding this mesoregion. Additionally, we investigated the species diversity and phenology of bumblebees on red clover (Trifolium pratense). Many authors made observations on bumblebees visiting red clover fields in different parts of Poland (Błażejewska et al. -
Pinyon Pine Mortality Alters Communities of Ground-Dwelling Arthropods
Western North American Naturalist 74(2), © 2014, pp. 162–184 PINYON PINE MORTALITY ALTERS COMMUNITIES OF GROUND-DWELLING ARTHROPODS Robert J. Delph1,2,6, Michael J. Clifford2,3, Neil S. Cobb2, Paulette L. Ford4, and Sandra L. Brantley5 ABSTRACT.—We documented the effect of drought-induced mortality of pinyon pine (Pinus edulis Engelm.) on com- munities of ground-dwelling arthropods. Tree mortality alters microhabitats utilized by ground-dwelling arthropods by increasing solar radiation, dead woody debris, and understory vegetation. Our major objectives were to determine (1) whether there were changes in community composition, species richness, and abundance of ground-dwelling arthro- pods associated with pinyon mortality and (2) whether specific habitat characteristics and microhabitats accounted for these changes. We predicted shifts in community composition and increases in arthropod diversity and abundance due to the presumed increased complexity of microhabitats from both standing dead and fallen dead trees. We found signifi- cant differences in arthropod community composition between high and low pinyon mortality environments, despite no differences in arthropod abundance or richness. Overall, 22% (51 taxa) of the arthropod community were identified as being indicators of either high or low mortality. Our study corroborates other research indicating that arthropods are responsive to even moderate disturbance events leading to changes in the environment. These arthropod responses can be explained in part due to the increase in woody debris and reduced canopy cover created by tree mortality. RESUMEN.—Documentamos el efecto de la mortalidad causada por la sequía del pino piñonero (Pinus edulis Engelm.) sobre comunidades de artrópodos subterráneos. Utilizamos tres variantes en el microhábitat de los artrópodos incrementando la radiación solar, desechos de madera muerta y vegetación baja. -
An Examination of Synchrony Between Insect Emergence and flowering in Rocky Mountain Meadows
Ecological Monographs, 81(3), 2011, pp. 469–491 Ó 2011 by the Ecological Society of America An examination of synchrony between insect emergence and flowering in Rocky Mountain meadows 1,3 2 JESSICA R. K. FORREST AND JAMES D. THOMSON 1Department of Ecology and Evolutionary Biology, University of Toronto, 25 Harbord St., Toronto, Ontario M5S 3G5 Canada 2Rocky Mountain Biological Laboratory, P.O. Box 519, Crested Butte, Colorado 81224 USA Abstract. One possible effect of climate change is the generation of a mismatch in the seasonal timing of interacting organisms, owing to species-specific shifts in phenology. Despite concerns that plants and pollinators might be at risk of such decoupling, there have been few attempts to test this hypothesis using detailed phenological data on insect emergence and flowering at the same localities. In particular, there are few data sets on pollinator flight seasons that are independent of flowering phenology, because pollinators are typically collected at flowers. To address this problem, we established standardized nesting habitat (trap nests) for solitary bees and wasps at sites along an elevational gradient in the Rocky Mountains, and monitored emergence during three growing seasons. We also recorded air temperatures and flowering phenology at each site. Using a reciprocal transplant experiment with nesting bees, we confirmed that local environmental conditions are the primary determinants of emergence phenology. We were then able to develop phenology models to describe timing of pollinator emergence or flowering, across all sites and years, as a function of accumulated degree-days. Although phenology of both plants and insects is well described by thermal models, the best models for insects suggest generally higher threshold temperatures for development or diapause termination than those required for plants. -
Simultaneous Percussion by the Larvae of a Stem-Nesting Solitary
JHR 81: 143–164 (2021) doi: 10.3897/jhr.81.61067 RESEARCH ARTICLE https://jhr.pensoft.net Simultaneous percussion by the larvae of a stem- nesting solitary bee – a collaborative defence strategy against parasitoid wasps? Andreas Müller1, Martin K. Obrist2 1 ETH Zurich, Institute of Agricultural Sciences, Biocommunication and Entomology, Schmelzbergstrasse 9/ LFO, 8092 Zurich, Switzerland 2 Swiss Federal Research Institute WSL, Biodiversity and Conservation Biol- ogy, 8903 Birmensdorf, Switzerland Corresponding author: Andreas Müller ([email protected]) Academic editor: Michael Ohl | Received 23 November 2020 | Accepted 7 February 2021 | Published 25 February 2021 http://zoobank.org/D10742E1-E988-40C1-ADF6-7F8EC24D6FC4 Citation: Müller A, Obrist MK (2021) Simultaneous percussion by the larvae of a stem-nesting solitary bee – a collaborative defence strategy against parasitoid wasps? Journal of Hymenoptera Research 81: 143–164. https://doi. org/10.3897/jhr.81.61067 Abstract Disturbance sounds to deter antagonists are widespread among insects but have never been recorded for the larvae of bees. Here, we report on the production of disturbance sounds by the postdefecating larva (“prepupa”) of the Palaearctic osmiine bee Hoplitis (Alcidamea) tridentata, which constructs linear series of brood cells in excavated burrows in pithy plant stems. Upon disturbance, the prepupa produces two types of sounds, one of which can be heard up to a distance of 2–3 m (“stroking sounds”), whereas the other is scarcely audible by bare ear (“tapping sounds”). To produce the stroking sounds, the prepupa rapidly pulls a horseshoe-shaped callosity around the anus one to five times in quick succession over the cocoon wall before it starts to produce tapping sounds by knocking a triangularly shaped callosity on the clypeus against the cocoon wall in long uninterrupted series of one to four knocks per second. -
Changes in the Summer Wild Bee Community Following a Bark Beetle Outbreak in a Douglas-Fir Forest
Environmental Entomology, XX(XX), 2020, 1–12 doi: 10.1093/ee/nvaa119 Pollinator Ecology and Management Research Changes in the Summer Wild Bee Community Following a Bark Beetle Outbreak in a Douglas-fir Forest Gabriel G. Foote,1,6,7 Nathaniel E. Foote,2 Justin B. Runyon,3 Darrell W. Ross,1,4 and Christopher J. Fettig5 1Forest Ecosystems and Society, Oregon State University, Corvallis, OR 97331, 2Forest and Rangeland Stewardship, Colorado State University, Fort Collins, CO 80523, 3Rocky Mountain Research Station, USDA Forest Service, 1648 South 7th Street, Bozeman, MT 59717, 4Present address: Department of Entomology, North Dakota State University, Fargo, ND 58108, 5Pacific Southwest Research Station, USDA Forest Service, 1731 Research Park Drive, Davis, CA 95618, 6Present address: Department of Entomology and Nematology, University of California, Davis, Davis, CA 95616, and 7Corresponding author, e-mail: [email protected] Subject Editor: Theresa Pitts-Singer Received 23 March 2020; Editorial decision 28 August 2020 Abstract AADate The status of wild bees has received increased interest following recent estimates of large-scale declines in their abundances across the United States. However, basic information is limited regarding the factors affecting wild bee communities in temperate coniferous forest ecosystems. To assess the early responses of bees to bark beetle AAMonth disturbance, we sampled the bee community of a Douglas-fir,Pseudotsuga menziesii (Mirb.), forest in western Idaho, United States during a Douglas-fir beetle,Dendroctonus pseudotsugae Hopkins (Coleoptera: Curculionidae), AAYear outbreak beginning in summer 2016. We resampled the area in summer 2018 following reductions in forest canopy cover resulting from mortality of dominant and codominant Douglas-fir.