Separating Electronic from Steric Effects in Ethene/Α-Olefin
Total Page:16
File Type:pdf, Size:1020Kb
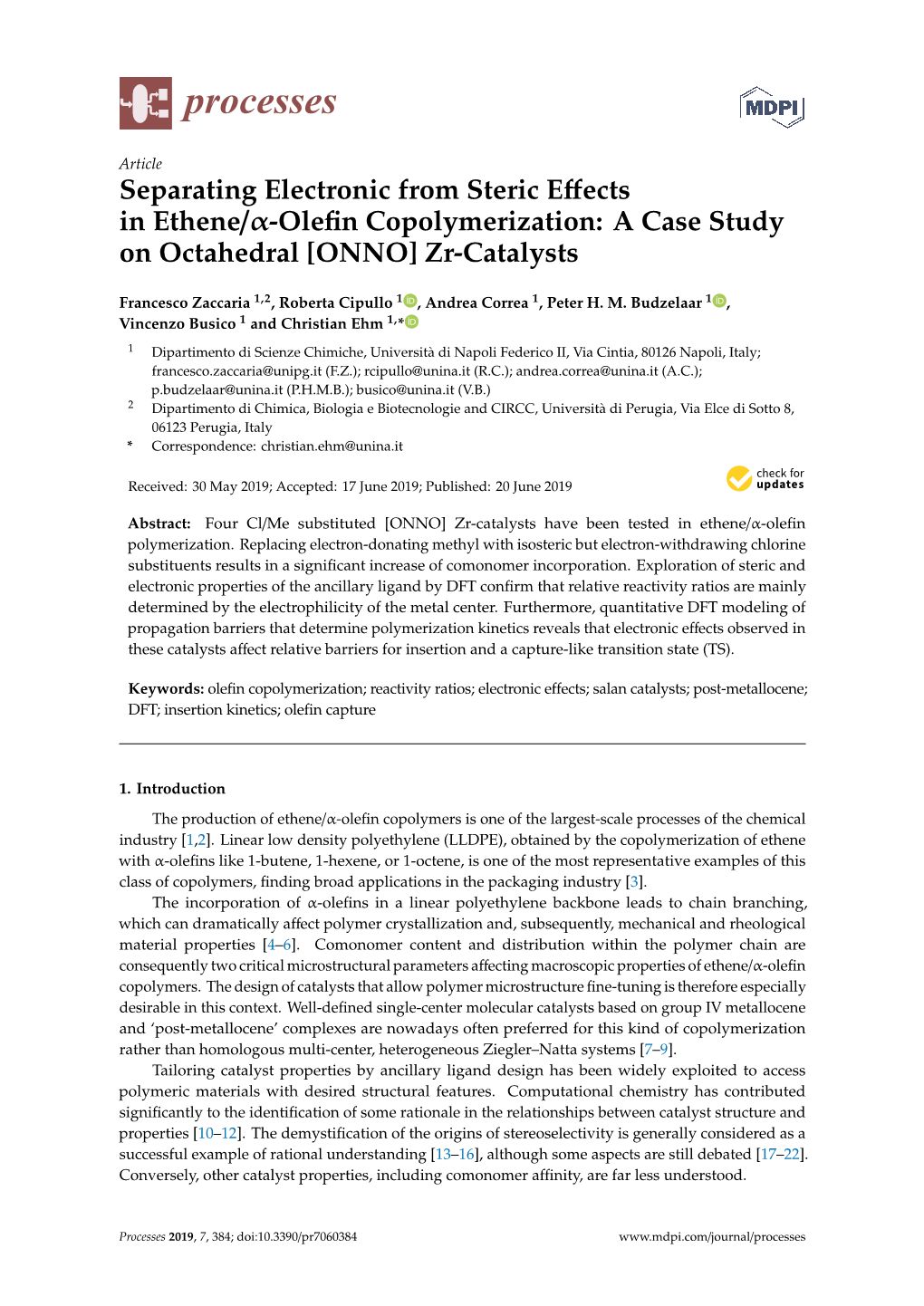
Load more
Recommended publications
-
Stabilizing a Different Cyclooctatetraene Stereoisomer
Stabilizing a different cyclooctatetraene stereoisomer Longfei Lia,b, Ming Leia,1, Yaoming Xieb, Henry F. Schaefer IIIb,1, Bo Chenc, and Roald Hoffmannc,1 aState Key Laboratory of Chemical Resource Engineering, Institute of Materia Medica, College of Science, Beijing University of Chemical Technology, Beijing 100029, People’s Republic of China; bCenter for Computational Quantum Chemistry, University of Georgia, Athens, GA 30602; and cDepartment of Chemistry and Chemical Biology, Cornell University, Ithaca, NY 14853-1301 Contributed by Roald Hoffmann, July 27, 2017 (sent for review June 6, 2017; reviewed by Henry Rzepa and Jay S. Siegel) An unconventional cis-cis-cis-trans or (Z,Z,Z,E) structure B of cyclo- (Z,Z,Z,E)-COT octatetraene (COT) is calculated to lie only 23 kcal/mol above the A Second COT Isomer. As did the above-cited authors, we located the well-known tub-shaped (Z,Z,Z,Z) isomer A; one example of this type (Z,Z,Z,E) isomer of COT (B) as a local minimum on its potential of structure is known. The barrier for B returning to A is small, energy surface. Throughout this paper, we use the ωB97X-D/cc- 3 kcal/mol. However, by suitable choice of substituents, the (Z,Z,Z,E) pVTZ (density functional theory method using the ωb97X-D func- isomer can be made to lie in energy below the tub-shaped structure. tional with correlation-consistent polarized valence triple-zeta basis Steric, clamping, and electronic strategies are proposed for achieving set) method of calculation (details are provided in SI Appendix) t B this. -
The Case of Sterimol Steric Parameters A
Conformational Effects on Physical-Organic Descriptors – the Case of Sterimol Steric Parameters A. V. Brethomé,† S. P. Fletcher†* and R. S. Paton†§* †Chemistry Research Laboratory, University of Oxford, Mansfield Road, Oxford OX1 3TA, UK §Department of Chemistry, Colorado State University, Fort Collins, Colorado 80523, USA Abstract Mathematical relationships which relate chemical structure with selectivity have provided quantitative insights underlying catalyst design and informing mechanistic studies. Flexible compounds, however, can adopt several distinct geometries and so can be challenging to describe using a single structure-based descriptor. How best to quantify the structural characteristics of an ensemble of structure poses both practical and technical difficulties. In this work we introduce an automated computational workflow which can be used to obtain multidimensional Sterimol parameters for a conformational ensemble of a given substituent from a single command. The Boltzmann-weighted Sterimol parameters obtained from this approach are shown to be useful in multivariate models of enantioselectivity, while the range of values from conformers within 3 kcal/mol of the most stable structure provides a visual way to capture a possible source of uncertainty arising in the resulting models. Implementing our approach requires no programming expertise and can be executed from within a graphical user interface using open-source programs. Introduction Steric effects are key nonbonding interactions influencing molecular conformation and -
Steric Effects Vs. Electron Delocalization
RSC Advances PAPER View Article Online View Journal | View Issue Steric effects vs. electron delocalization: a new look into the stability of diastereomers, conformers Cite this: RSC Adv.,2021,11, 20691 and constitutional isomers† Sopanant Datta and Taweetham Limpanuparb * A quantum chemical investigation of the stability of compounds with identical formulas was carried out on 23 classes of compounds made of C, N, P, O and S atoms as core structures and halogens H, F, Cl, Br and I as Received 13th April 2021 substituents. All possible structures were generated and investigated by quantum mechanical methods. The Accepted 24th May 2021 prevalence of a formula in which its Z configuration, gauche conformation or meta isomer is the most stable DOI: 10.1039/d1ra02877d form is calculated and discussed. Quantitative and qualitative models to explain the stability of the 23 classes rsc.li/rsc-advances of halogenated compounds were also proposed. hyperconjugation in a similar vein to the ethane case,17,18 but Creative Commons Attribution-NonCommercial 3.0 Unported Licence. 1. Introduction the reasoning underlying the preference for meta isomer is still Steric effects, non-bonded interactions leading to avoidance of lacking. spatial congestion of atoms or groups, are oen the central In addition to carbon-backbone compounds in Table 1, there theme in the discussion of stability of diastereomers, are many experimental and theoretical studies for other back- 21 ] 22,23 ] 24 conformers and constitutional isomers. Reasoning based on bones discussed in this paper, namely, C3, C N, C P, 25–31 32 33,34 35 36,37 38–40 41 steric effects is relatively intuitive and gives rise to a generally N]N, N]P, P]P, C–N, C–P, C–O, C–S, N– 38,42–44 38,43 38,43,45,46 38,42,47 47 48,49 50 accepted rule of thumb that an E conguration, anti conformer N, N–P, P–P, N–O, N–S, P–O, P–S, O– 38,42,51,52 53,54 38,55 and para isomer in diastereomers, conformational and consti- O, O–S, and S–S. -
Computational Measurement of Steric Effects: the Size of Organic Substituents Computed by Ligand Repulsive Energies
J. Org. Chem. 1999, 64, 7707-7716 7707 Computational Measurement of Steric Effects: the Size of Organic Substituents Computed by Ligand Repulsive Energies David P. White,*,1 Jan C. Anthony,2 and Ademola O. Oyefeso2 Department of Chemistry, University of North CarolinasWilmington, 601 South College Rd., Wilmington, North Carolina 28403-3297 Received December 8, 1998 Ligand repulsive energies, ER, have been demonstrated to provide reliable steric parameters for ligands in organometallic systems. ER values have now been computed for 167 different organic substituents. Three different fragments were employed for the calculation of the ligand repulsive energies: CH3,CH2COOH, and Cr(CO)5 . All compounds were modeled using molecular mechanics. Two different force fields were employed: Allinger’s MMP2 and Rappe´’s Universal Force Field (UFF). Both molecular dynamics and stochastic mechanics were used to determine the lowest energy conformer for each species. Steric sizes were compared against standard steric measures in organic chemistry: Taft-Dubois steric parameter, E′S, A-values, cone angles, θ, and solid angles, ΩS. Good correlations between ER and the model-based steric measures (θ and ΩS) were found. Experimental- based measures, E′S and A-values, showed a mix of steric and electronic effects. On the basis of these correlations, the use of CH3,CH2COOH, and Cr(CO)5 fragments for steric size quantification was critically examined. Introduction outlined above would respond identically to steric effects. Therefore, Dubois chose a single standard: the acid- Over 100 years ago the importance of the size of a catalyzed esterification of carboxylic acids in methanol substituent in determining the rate of a given transfor- at 40 °C. -
Impact of Rigidity on Molecular Self-Assembly
Impact of Rigidity on Molecular Self-Assembly Ella M. King1,2, Matthew A. Gebbie1,3, Nicholas A. Melosh1,4* *E-mail: [email protected] 1Geballe Laboratory for Advanced Materials, Stanford University, Stanford, CA 94305 2Department of Physics, Harvard University, Cambridge, MA 02138 3Department of Chemical and Biological Engineering, University of Wisconsin-Madison, Madison, WI 53706 4Department of Materials Science and Engineering, Stanford University, Stanford, CA 94305 Abstract Rigid, cage-like molecules, like diamondoids, show unique self-assembly behavior, such as templating 1-D nanomaterial assembly via pathways that are typically blocked for such bulky substituents. We investigate molecular forces between diamondoids to explore why molecules with high structural rigidity exhibit these novel assembly pathways. The rigid nature of diamondoids significantly lowers configurational entropy, and we hypothesize that this influences molecular interaction forces. To test this concept, we calculated the distance-dependent impact of entropy on assembly using molecular dynamics simulations. To isolate pairwise entropic and enthalpic contributions to assembly, we considered pairs of molecules in a thermal bath, fixed at set intermolecular separations but otherwise allowed to freely move. By comparing diamondoids to linear alkanes, we draw out the impact of rigidity on the entropy and enthalpy of pairwise interactions. We find that linear alkanes actually exhibit stronger van der Waals interactions than diamondoids at contact, because the bulky structure of diamondoids induces larger net atomic separations. Yet, we also find that diamondoids pay lower entropic penalties when assembling into contact pairs. Thus, the cage-like shape of diamondoids introduces an enthalpic penalty at contact, but the penalty is counterbalanced by entropic effects. -
One-Pot Synthesis of CF3-Substituted Pyrazolines/Pyrazoles from Electron
FULL PAPER DOI: 10.1002/ejoc.201301852 One-Pot Synthesis of CF3-Substituted Pyrazolines/Pyrazoles from Electron- Deficient Alkenes/Alkynes and CF3CHN2 Generated in situ: Optimized Synthesis of Tris(trifluoromethyl)pyrazole Evgeniy Y. Slobodyanyuk,[a] Olexiy S. Artamonov,[b] Oleg V. Shishkin,[c] and Pavel K. Mykhailiuk*[a,d] Keywords: Synthetic methods / Nitrogen heterocycles / Fluorine / Multicomponent reactions / Cycloaddition The [3+2] cycloaddition of CF3CHN2, generated in situ, with three-component reaction between CF3CH2NH2·HCl, electron-deficient alkenes/alkynes affords CF3-substituted NaNO2, and the substrate proceeds at room temperature in pyrazolines/pyrazoles in quantitative yields. The one-pot dichloromethane/water. Introduction In 1968, Atherton and Fields prepared CF3-substituted Pyrazolines play an important role in drug discovery and pyrazolines by [3+2] cycloaddition between CF3CHN2 and organic synthesis: they exhibit a broad spectrum of bio- alkenes.[8] The transformation, however, was technically logical activities (Figure 1),[1,2] and serve as precursors to challenging, because it required (i) generation of the [3] [4] [5] [9,10] cyclopropanes, pyrazoles, and diamines. Considering toxic, potentially explosive gas CF3CHN2 from [6,7] that more than 45 drugs contain the CF3 group, CF3- CF3CH2NH2·HCl and NaNO2 and (ii) purification of the substituted pyrazolines look to be promising molecules for reagent by two vacuum distillations at –80 and –196 °C.[11] medicinal chemists. Therefore, this method has had very little practical applica- Figure 1. Bioactive pyrazolines.[1,2] [12,13] [a] Organic Chemistry Department, Taras Shevchenko National tion so far. In this context, we have developed a safe, University of Kyiv, one-pot procedure to prepare CF3-substituted pyrazolines Volodymyrska 64, Kyiv 01601, Ukraine without isolation of the dangerous CF CHN . -
PHYSICAL ORGANIC Chemistryl
PHYSICAL ORGANIC CHEMISTRYl By EDWARD R. THORNTON Department of Chemistry, University of Pennsylvania, Philadelphia, Pennsylvania The author, who considers himself to have interests typical of a physical organic chemist, found about 2300 papers published during the last year that were of special interest to him. He confesses that he could not possibly read them all and hopes that those whose work may have been left out by neces sity or oversight will understand the problems involved. Most of this review discusses secondary and solvent isotope effects. Mention is also made of recent developments in quantum chemistry, es pecially in the qualitative discussion of orbital symmetry as a determining factor for certain reaction pathways. It cannot be claimed that enough space was available for comprehensive coverage of even these restricted topics. SECONDARY DEUTERIUM ISOTOPE EFFECTS A great deal of interesting information on secondary isotope effects has become available since the comprehensive review by Halevi (1) in 1963. Advances have been made in both experimental studies and theoretical un derstanding. Some of this new work, along with the present author's inter pretation of secondary isotope effects, will be discussed. Origin of secondary isotope effects.-A great deal of difficulty occurs in attempting to describe the causes of secondary isotope effects. Nevertheless, as far as the author knows, there is no case where an appropriate statistical treatment of isotope effects [Bigeleisen & Wolfsberg (2), Melander (3), Thornton (4)] does not adequately describe experiment. Even in terms of isotopic effects of the order of a few per cent, this theory and the approxima tions it uses are very precise. -
Physical Organic Chemistry
CHM 8304 Physical Organic Chemistry Thermodynamics and kinetics Outline: Isotope effects • see section 8.1 of A&D – experimental approach – primary isotope effect – secondary isotope effect – equilibrium isotope effect – solvent isotope effect – heavy atom isotope effects 2 Thermodynamics and kinetics 1 CHM 8304 Measurement of an isotope effect • performed to determine if a bond changes in a certain way during the rate-limiting step • expressed as a ratio whose numerator is the rate constant measured for the naturally abundant isotope and the denominator is the rate constant measured for the varied isotope – e.g. kH/kD 3 Types of isotope effects • kinetic isotope effects (kie): result from a change in the rate constant of a reaction : – normal effect: ratio > 1 – inverse effect: ratio < 1 – primary isotope effect: when the isotopically substituted bond is cleaved during the rate-limiting step – secondary isotope effect : attributable to a change of hybridation state, not cleavage of bonds • equilibrium isotope effects: result from displacement of an equilibrium 4 Thermodynamics and kinetics 2 CHM 8304 Origin of isotope effects • the origin of all isotope effects is a difference in the frequency of vibrational modes of a substituted molecule with respect to an unsubstituted molecule • it is these vibrational modes that principally affect the shape of the potential energy well on an energy surface 5 Zero point energy • zero point energy (ZPE) is the energy level of the vibrational ground state for most molecules at ambient temperature • -
The Interplay of Steric and Electronic Factors Affecting Geometrical Isomerism of Dtaryl Ket~M~Ne Derivatives
Tctrahcxlron, 1960, Vol. 9, pp. 210 co 229. Pcrgamon Press Ltd. Printed in Mxthcrn Ireland THE INTERPLAY OF STERIC AND ELECTRONIC FACTORS AFFECTING GEOMETRICAL ISOMERISM OF DTARYL KET~M~NE DERIVATIVES P. A. S. SMITHand E. P. ANTONIADES* Dept. of Chemistry, University of Michigan, Ann Arbor, Michigan AbslracG-The evidence bearing on the mechanism of the Schmidt reaction with ketones is critically reexamined. It is shown that dehydration of an initially formed azid~hydrin (I) to a ketimino- di~nium ion (II), which may or may not equilibrate between its geometrj~lly isomeric forms (TIa or b), can account for the various reported ratios of isomer2 amides produced from unsymmetri~l ketones. The previously anomalous behavior of w&-substituted diary1 ketones can be resolved by taking into account the influence of conjugative effects on the preferred rotational positions. The ratios of amides produced from a series of 4.kylbenzophenones by both the Schmidt reaction and oximation pfus Beckmann r~~angement have been dete~in~ at different temperatures; there are appreciabIe differences in the product ratios and their temperature coefficients from the two reactions. IN 1948 one of us proposed’ a mechanism for the Schmidt reaction2 between hydrogen azide and ketones, which in the ensuing decade has been the basis for most discussion of the reaction. So much pertinent excremental investigation and theoretiGa1 discus- sion has since been published that a reconsideration of the overall picture seems justified at this time. ‘The principal reaction products of ketones and hydrogen azide are amides, of which a single compound is produced from symmetri~l ketones, but a pair of isomers may be produced in unequal amounts from unsymmetrical ketones. -
1,3-Dipolar Cycloadditions of Nitrones and Nitrile Oxides. Yau-Min Chang Louisiana State University and Agricultural & Mechanical College
Louisiana State University LSU Digital Commons LSU Historical Dissertations and Theses Graduate School 1975 1,3-Dipolar Cycloadditions of Nitrones and Nitrile Oxides. Yau-min Chang Louisiana State University and Agricultural & Mechanical College Follow this and additional works at: https://digitalcommons.lsu.edu/gradschool_disstheses Recommended Citation Chang, Yau-min, "1,3-Dipolar Cycloadditions of Nitrones and Nitrile Oxides." (1975). LSU Historical Dissertations and Theses. 2865. https://digitalcommons.lsu.edu/gradschool_disstheses/2865 This Dissertation is brought to you for free and open access by the Graduate School at LSU Digital Commons. It has been accepted for inclusion in LSU Historical Dissertations and Theses by an authorized administrator of LSU Digital Commons. For more information, please contact [email protected]. INFORMATION TO USERS This material was produced from a microfilm copy of the original document. While the most advanced technological means to photograph and reproduce this document have been used, the quality is heavily dependent upon the quality of the original submitted. The following explanation of techniques is provided to help you understand markings or patterns which may appear on this reproduction. 1. The sign or "target" for pages apparently lacking from the document photographed is "Missing Page(s)". If it was possible to obtain the missing page(s) or section, they are spliced into the film along with adjacent pages. This may have necessitated cutting thru an image and duplicating adjacent pages to insure you complete continuity. 2. When an image on the film is obliterated with a large round black mark, it is an indication that the photographer suspected that the copy may have moved during exposure and thus cause a blurred image. -
Physical Organic Chemistry 2013-2014
Université Catholique de Louvain - COURSES DESCRIPTION FOR 2013-2014 - LCHM2143 LCHM2143 Physical organic chemistry 2013-2014 3.0 credits 22.5 h + 7.5 h 1q Teacher(s) : Robiette Raphaël ; Riant Olivier ; Language : Français Place of the course Louvain-la-Neuve Main themes : This course is aimed to a synthesis of various notions related to physical organic chemistry and already introduced in the various courses from the preceding years. It also gives an introduction to some selected physico-chemical tools used in the elucidation of reaction mechanisms in organic chemistry. The main themes are: -Structure -activity relationships in organic chemistry -Electronic and sterics effects -Influence of the reaction media in organic chemistry -Stereoelectronic effects in organic chemistry Aims : The aim of this course is to introduce important notions and concepts selected in the field of physical organic chemistry. One of the goals of this course is to use those notions for a better understanding of reaction mechanisms in organic chemistry, the structure of reaction intermediates and transition states, and a deeper understanding of the molecular interactions which can influence chemical reactivity. The contribution of this Teaching Unit to the development and command of the skills and learning outcomes of the programme(s) can be accessed at the end of this sheet, in the section entitled “Programmes/courses offering this Teaching Unit”. Content : 1-Structure-activity relationships: Free energy relationships. Quantitative estimation of electronic effects (Hammett and Yukawa- Tsuno equations). Application to organic reaction mechanisms. Sigma scales. Deviations to the Hammett equation. Physico- chemical methods for the measurements of the sigma parameters. -
Using Elementary Reactions to Model Growth
Using elementary Reactions to Model Growth Processes of Polyaromatic Hydrocarbons under Pyrolysis Conditions of Light Feedstocks Karen Hemelsoet, Veronique van Speybroeck, Kevin M. van Geem, Guy B Marin, Michel Waroquier To cite this version: Karen Hemelsoet, Veronique van Speybroeck, Kevin M. van Geem, Guy B Marin, Michel Waroquier. Using elementary Reactions to Model Growth Processes of Polyaromatic Hydrocarbons under Pyrolysis Conditions of Light Feedstocks. Molecular Simulation, Taylor & Francis, 2008, 34 (02), pp.193-199. 10.1080/08927020801930588. hal-00515027 HAL Id: hal-00515027 https://hal.archives-ouvertes.fr/hal-00515027 Submitted on 4 Sep 2010 HAL is a multi-disciplinary open access L’archive ouverte pluridisciplinaire HAL, est archive for the deposit and dissemination of sci- destinée au dépôt et à la diffusion de documents entific research documents, whether they are pub- scientifiques de niveau recherche, publiés ou non, lished or not. The documents may come from émanant des établissements d’enseignement et de teaching and research institutions in France or recherche français ou étrangers, des laboratoires abroad, or from public or private research centers. publics ou privés. For Peer Review Only Using elementary Reactions to Model Growth Processes of Polyaromatic Hydrocarbons under Pyrolysis Conditions of Light Feedstocks Journal: Molecular Simulation/Journal of Experimental Nanoscience Manuscript ID: GMOS-2007-0171.R1 Journal: Molecular Simulation Date Submitted by the 09-Jan-2008 Author: Complete List of Authors: Hemelsoet,