View and Expressed As the Number of Mhspb1 Positive Cells Over Total Cells
Total Page:16
File Type:pdf, Size:1020Kb
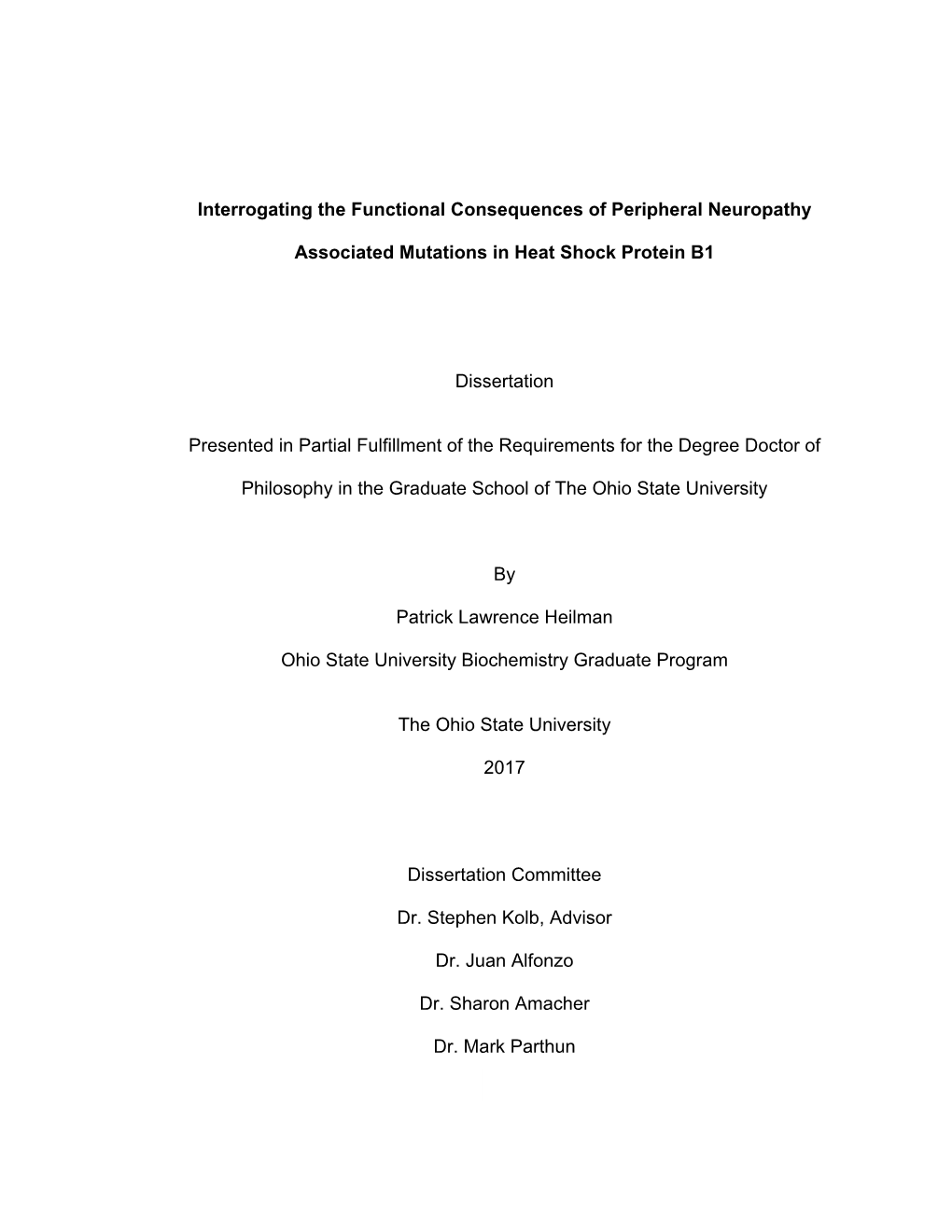
Load more
Recommended publications
-
Computational Genome-Wide Identification of Heat Shock Protein Genes in the Bovine Genome [Version 1; Peer Review: 2 Approved, 1 Approved with Reservations]
F1000Research 2018, 7:1504 Last updated: 08 AUG 2021 RESEARCH ARTICLE Computational genome-wide identification of heat shock protein genes in the bovine genome [version 1; peer review: 2 approved, 1 approved with reservations] Oyeyemi O. Ajayi1,2, Sunday O. Peters3, Marcos De Donato2,4, Sunday O. Sowande5, Fidalis D.N. Mujibi6, Olanrewaju B. Morenikeji2,7, Bolaji N. Thomas 8, Matthew A. Adeleke 9, Ikhide G. Imumorin2,10,11 1Department of Animal Breeding and Genetics, Federal University of Agriculture, Abeokuta, Nigeria 2International Programs, College of Agriculture and Life Sciences, Cornell University, Ithaca, NY, 14853, USA 3Department of Animal Science, Berry College, Mount Berry, GA, 30149, USA 4Departamento Regional de Bioingenierias, Tecnologico de Monterrey, Escuela de Ingenieria y Ciencias, Queretaro, Mexico 5Department of Animal Production and Health, Federal University of Agriculture, Abeokuta, Nigeria 6Usomi Limited, Nairobi, Kenya 7Department of Animal Production and Health, Federal University of Technology, Akure, Nigeria 8Department of Biomedical Sciences, Rochester Institute of Technology, Rochester, NY, 14623, USA 9School of Life Sciences, University of KwaZulu-Natal, Durban, 4000, South Africa 10School of Biological Sciences, Georgia Institute of Technology, Atlanta, GA, 30032, USA 11African Institute of Bioscience Research and Training, Ibadan, Nigeria v1 First published: 20 Sep 2018, 7:1504 Open Peer Review https://doi.org/10.12688/f1000research.16058.1 Latest published: 20 Sep 2018, 7:1504 https://doi.org/10.12688/f1000research.16058.1 Reviewer Status Invited Reviewers Abstract Background: Heat shock proteins (HSPs) are molecular chaperones 1 2 3 known to bind and sequester client proteins under stress. Methods: To identify and better understand some of these proteins, version 1 we carried out a computational genome-wide survey of the bovine 20 Sep 2018 report report report genome. -
Identification of the Binding Partners for Hspb2 and Cryab Reveals
Brigham Young University BYU ScholarsArchive Theses and Dissertations 2013-12-12 Identification of the Binding arP tners for HspB2 and CryAB Reveals Myofibril and Mitochondrial Protein Interactions and Non- Redundant Roles for Small Heat Shock Proteins Kelsey Murphey Langston Brigham Young University - Provo Follow this and additional works at: https://scholarsarchive.byu.edu/etd Part of the Microbiology Commons BYU ScholarsArchive Citation Langston, Kelsey Murphey, "Identification of the Binding Partners for HspB2 and CryAB Reveals Myofibril and Mitochondrial Protein Interactions and Non-Redundant Roles for Small Heat Shock Proteins" (2013). Theses and Dissertations. 3822. https://scholarsarchive.byu.edu/etd/3822 This Thesis is brought to you for free and open access by BYU ScholarsArchive. It has been accepted for inclusion in Theses and Dissertations by an authorized administrator of BYU ScholarsArchive. For more information, please contact [email protected], [email protected]. Identification of the Binding Partners for HspB2 and CryAB Reveals Myofibril and Mitochondrial Protein Interactions and Non-Redundant Roles for Small Heat Shock Proteins Kelsey Langston A thesis submitted to the faculty of Brigham Young University in partial fulfillment of the requirements for the degree of Master of Science Julianne H. Grose, Chair William R. McCleary Brian Poole Department of Microbiology and Molecular Biology Brigham Young University December 2013 Copyright © 2013 Kelsey Langston All Rights Reserved ABSTRACT Identification of the Binding Partners for HspB2 and CryAB Reveals Myofibril and Mitochondrial Protein Interactors and Non-Redundant Roles for Small Heat Shock Proteins Kelsey Langston Department of Microbiology and Molecular Biology, BYU Master of Science Small Heat Shock Proteins (sHSP) are molecular chaperones that play protective roles in cell survival and have been shown to possess chaperone activity. -
45–54 Physical Location of Genes
Rocz. Nauk. Zoot., T. 44, z. 1 (2017) 45–54 PHYSICAL LOCATION OF GENES ENCODING SMALL HEAT SHOCK PROTEINS IN THE SUIDAE GENOMES* * Barbara Danielak-Czech1 , Anna Kozubska-Sobocińska1 , Marek Babicz2 1National Research Institute of Animal Production, Department of Animal Genomics and Molecular Biology, 32-083 Balice n. Kraków, Poland 2University of Life Sciences in Lublin, Faculty of Biology, Animal Sciences and Bioeconomy, Akademicka 13, 20-950 Lublin, Poland The subject of the studies carried out was physical mapping of the HSPB1, HSPB2, CRY- AB (alternative name HSPB5), HSPB6 and HSPB8 genes from the family of small heat shock protein genes (HSPB) on chromosomes of the domestic pig (Sus scrofa domestica) and European wild pig (Sus scrofa scrofa). The application of FISH technique with pro- bes derived from porcine BAC clones: CH242-237N5, CH242-333E2, CH242-173G9 and CH242-102C8 made it possible to determine the location of the studied genes, respectively, in 3p15, 9p21, 6q12 and 14q21 genome regions of domestic and wild pigs. The physical localization of HSPB genes allowed assigning these loci to the linkage and syntenic groups of genes in Suidae. Precise, molecular and cytogenetic identification of genes responsible for resistance to stress and disease, and determining meat production is essential for the genetic selection effects, aimed to reduce mortality causing significant economic loss in animal production. The studies performed may help to elucidate the role of the HSPB genes in protection against pathogenic or environmental stress, affecting pigs’ survivability and meat quality. Key words: Suidae, FISH, HSPB genes, muscle development, meat quality Small heat shock proteins (HSPB) are the smallest, most variable in size, class of the multigene heat shock protein (HSP) family, having molecular masses ranging approximately from 15 to 30 kDa and the α-crystallin domains (~85 amino acids residues) in the highly conserved C-terminal protein regions. -
1 Supporting Information for a Microrna Network Regulates
Supporting Information for A microRNA Network Regulates Expression and Biosynthesis of CFTR and CFTR-ΔF508 Shyam Ramachandrana,b, Philip H. Karpc, Peng Jiangc, Lynda S. Ostedgaardc, Amy E. Walza, John T. Fishere, Shaf Keshavjeeh, Kim A. Lennoxi, Ashley M. Jacobii, Scott D. Rosei, Mark A. Behlkei, Michael J. Welshb,c,d,g, Yi Xingb,c,f, Paul B. McCray Jr.a,b,c Author Affiliations: Department of Pediatricsa, Interdisciplinary Program in Geneticsb, Departments of Internal Medicinec, Molecular Physiology and Biophysicsd, Anatomy and Cell Biologye, Biomedical Engineeringf, Howard Hughes Medical Instituteg, Carver College of Medicine, University of Iowa, Iowa City, IA-52242 Division of Thoracic Surgeryh, Toronto General Hospital, University Health Network, University of Toronto, Toronto, Canada-M5G 2C4 Integrated DNA Technologiesi, Coralville, IA-52241 To whom correspondence should be addressed: Email: [email protected] (M.J.W.); yi- [email protected] (Y.X.); Email: [email protected] (P.B.M.) This PDF file includes: Materials and Methods References Fig. S1. miR-138 regulates SIN3A in a dose-dependent and site-specific manner. Fig. S2. miR-138 regulates endogenous SIN3A protein expression. Fig. S3. miR-138 regulates endogenous CFTR protein expression in Calu-3 cells. Fig. S4. miR-138 regulates endogenous CFTR protein expression in primary human airway epithelia. Fig. S5. miR-138 regulates CFTR expression in HeLa cells. Fig. S6. miR-138 regulates CFTR expression in HEK293T cells. Fig. S7. HeLa cells exhibit CFTR channel activity. Fig. S8. miR-138 improves CFTR processing. Fig. S9. miR-138 improves CFTR-ΔF508 processing. Fig. S10. SIN3A inhibition yields partial rescue of Cl- transport in CF epithelia. -
Proteomic and Metabolomic Analyses of Mitochondrial Complex I-Deficient
THE JOURNAL OF BIOLOGICAL CHEMISTRY VOL. 287, NO. 24, pp. 20652–20663, June 8, 2012 © 2012 by The American Society for Biochemistry and Molecular Biology, Inc. Published in the U.S.A. Proteomic and Metabolomic Analyses of Mitochondrial Complex I-deficient Mouse Model Generated by Spontaneous B2 Short Interspersed Nuclear Element (SINE) Insertion into NADH Dehydrogenase (Ubiquinone) Fe-S Protein 4 (Ndufs4) Gene*□S Received for publication, November 25, 2011, and in revised form, April 5, 2012 Published, JBC Papers in Press, April 25, 2012, DOI 10.1074/jbc.M111.327601 Dillon W. Leong,a1 Jasper C. Komen,b1 Chelsee A. Hewitt,a Estelle Arnaud,c Matthew McKenzie,d Belinda Phipson,e Melanie Bahlo,e,f Adrienne Laskowski,b Sarah A. Kinkel,a,g,h Gayle M. Davey,g William R. Heath,g Anne K. Voss,a,h René P. Zahedi,i James J. Pitt,j Roman Chrast,c Albert Sickmann,i,k Michael T. Ryan,l Gordon K. Smyth,e,f,h b2 a,h,m,n3 David R. Thorburn, and Hamish S. Scott Downloaded from From the aMolecular Medicine Division, gImmunology Division, and eBioinformatics Division, Walter and Eliza Hall Institute of Medical Research, Parkville, Victoria 3052, Australia, the bMurdoch Childrens Research Institute, Royal Children’s Hospital and Department of Paediatrics, University of Melbourne, Parkville, Victoria 3052, Australia, the cDépartement de Génétique Médicale, Université de Lausanne, 1005 Lausanne, Switzerland, the dCentre for Reproduction and Development, Monash Institute of Medical Research, Clayton, Victoria 3168, Australia, the hDepartment of Medical Biology -
Recombinant Human Hspb2 (C-6His) Derived from E.Coli
Catalog # CH63 Recombinant Human HspB2 (C-6His) Derived from E.coli Recombinant Human Heat Shock Protein Beta-2 is produced by our E.coli expression system and the target gene encoding Met1-Pro182 is expressed with a 6His tag at the C-terminus. DESCRIPTION Accession Q16082 Known as Heat shock protein beta-2;HspB2;DMPK-binding protein;MKBP; Mol Mass 21.3kDa AP Mol Mass 22-25kDa, reducing conditions. QUALITY CONTROL Purity Greater than 95% as determined by reducing SDS-PAGE. Endotoxin Less than 0.1 ng/µg (1 EU/µg) as determined by LAL test. FORMULATION Lyophilized from a 0.2 μm filtered solution of 10mM Tris-HCl, 150mM NaCl, 1mM EDTA, pH 8.0. Always centrifuge tubes before opening. Do not mix by vortex or pipetting. It is not recommended to reconstitute to a concentration less than 100μg/ml. RECONSTITUTION Dissolve the lyophilized protein in distilled water. Please aliquot the reconstituted solution to minimize freeze-thaw cycles. The product is shipped at ambient temperature. SHIPPING Upon receipt, store it immediately at the temperature listed below. Lyophilized protein should be stored at < -20°C, though stable at room temperature for 3 weeks. STORAGE Reconstituted protein solution can be stored at 4-7°C for 2-7 days. Aliquots of reconstituted samples are stable at < -20°C for 3 months. Heat shock protein beta-2(HSPB2) is a protein that in humans is encoded by the HSPB2 gene. HSPB2 belongs to the superfamily of small heat-shock proteins containing a conservative alpha-crystallin domain at the C-terminal part of the molecule. -
Supplementary Materials
Supplementary materials Supplementary Table S1: MGNC compound library Ingredien Molecule Caco- Mol ID MW AlogP OB (%) BBB DL FASA- HL t Name Name 2 shengdi MOL012254 campesterol 400.8 7.63 37.58 1.34 0.98 0.7 0.21 20.2 shengdi MOL000519 coniferin 314.4 3.16 31.11 0.42 -0.2 0.3 0.27 74.6 beta- shengdi MOL000359 414.8 8.08 36.91 1.32 0.99 0.8 0.23 20.2 sitosterol pachymic shengdi MOL000289 528.9 6.54 33.63 0.1 -0.6 0.8 0 9.27 acid Poricoic acid shengdi MOL000291 484.7 5.64 30.52 -0.08 -0.9 0.8 0 8.67 B Chrysanthem shengdi MOL004492 585 8.24 38.72 0.51 -1 0.6 0.3 17.5 axanthin 20- shengdi MOL011455 Hexadecano 418.6 1.91 32.7 -0.24 -0.4 0.7 0.29 104 ylingenol huanglian MOL001454 berberine 336.4 3.45 36.86 1.24 0.57 0.8 0.19 6.57 huanglian MOL013352 Obacunone 454.6 2.68 43.29 0.01 -0.4 0.8 0.31 -13 huanglian MOL002894 berberrubine 322.4 3.2 35.74 1.07 0.17 0.7 0.24 6.46 huanglian MOL002897 epiberberine 336.4 3.45 43.09 1.17 0.4 0.8 0.19 6.1 huanglian MOL002903 (R)-Canadine 339.4 3.4 55.37 1.04 0.57 0.8 0.2 6.41 huanglian MOL002904 Berlambine 351.4 2.49 36.68 0.97 0.17 0.8 0.28 7.33 Corchorosid huanglian MOL002907 404.6 1.34 105 -0.91 -1.3 0.8 0.29 6.68 e A_qt Magnogrand huanglian MOL000622 266.4 1.18 63.71 0.02 -0.2 0.2 0.3 3.17 iolide huanglian MOL000762 Palmidin A 510.5 4.52 35.36 -0.38 -1.5 0.7 0.39 33.2 huanglian MOL000785 palmatine 352.4 3.65 64.6 1.33 0.37 0.7 0.13 2.25 huanglian MOL000098 quercetin 302.3 1.5 46.43 0.05 -0.8 0.3 0.38 14.4 huanglian MOL001458 coptisine 320.3 3.25 30.67 1.21 0.32 0.9 0.26 9.33 huanglian MOL002668 Worenine -
Consequences of Disease-Causing Small Heat Shock Protein Mutations on ARE-Mediated
Consequences of disease-causing small heat shock protein mutations on ARE-mediated mRNA decay Senior Honors Thesis By Nicole Naiman Undergraduate Biomedical Science Major School of Allied Medical Professions The Ohio State University 2011 Choose an item. Committee: Dr. Stephen Kolb, M.D., Ph.D, Advisor Dr. Daniel Battle, Ph.D. Dr. Margaret Teaford, Ph.D. Copyright by Nicole Naiman 2011 ii Abstract Motor neuron diseases (MNDs) are neurodegenerative diseases that involve loss of motor neurons in the brain and spinal cord. MNDs are debilitating and often fatal. Distal hereditary motor neuropathies (dHMNs) are a category of MND characterized by progressive, distal weakness without loss of sensation. The primary focus of our laboratory is to understand the functional consequences of mutations in small heat shock proteins (sHSPs) that result in dHMN. sHSPs comprise a family of 10 homologous proteins that are characterized by a central alpha-crystallin domain, are expressed ubiquitously, serve neuroprotective functions, and are upregulated by cell stress. To date, mutations in three sHSPs: HSPB1, HSPB3 and HSPB8, have been associated with dHMN. These mutations include HSPB1(R136W) and HSPB3(R7S). We propose that mutations reported in these proteins affect the same cellular pathway because they all lead to the same clinical phenotype and loss of motor neurons. HSPB1 is the best characterized sHSP and is required for AU-rich element (ARE)-dependent mRNA decay. AREs are adenosine and uridine rich regions that are present in the 3’ untranslated region of a subset of mRNAs that signal for their rapid decay. We hypothesize that dHMN-associated mutations result in dysregulation of this critical mRNA decay pathway, and that mutations in HSPB1 and in HSPB3 result in an increased half-life of ARE-containing mRNAs. -
Expansion De Triplets CTG Et Arrêt Prolifératif Précoce Des Myoblastes DM1 Erwan Gasnier
Expansion de triplets CTG et arrêt prolifératif précoce des myoblastes DM1 Erwan Gasnier To cite this version: Erwan Gasnier. Expansion de triplets CTG et arrêt prolifératif précoce des myoblastes DM1. Biochimie, Biologie Moléculaire. Université Pierre et Marie Curie - Paris VI, 2012. Français. NNT : 2012PAO66195. tel-00829312 HAL Id: tel-00829312 https://tel.archives-ouvertes.fr/tel-00829312 Submitted on 3 Jun 2013 HAL is a multi-disciplinary open access L’archive ouverte pluridisciplinaire HAL, est archive for the deposit and dissemination of sci- destinée au dépôt et à la diffusion de documents entific research documents, whether they are pub- scientifiques de niveau recherche, publiés ou non, lished or not. The documents may come from émanant des établissements d’enseignement et de teaching and research institutions in France or recherche français ou étrangers, des laboratoires abroad, or from public or private research centers. publics ou privés. THESE DE DOCTORAT DE L’UNIVERSITE PIERRE et MARIE CURIE École doctorale Complexité du Vivant (ED515) Présentée par Mr Erwan GASNIER Pour obtenir le grade de Docteur de l’université PARIS VI Spécialité Biologie Moléculaire et Cellulaire Sujet de thèse : EXPANSION DE TRIPLETS CTG ET ARRET PROLIFERATIF PRECOCE DES MYOBLASTES DM1 Thèse dirigée par : Dr FURLING Denis Soutenue le 13 Avril 2012 Devant le jury composé de : Pr FRIGUET Bertrand Président/Examinateur Dr BASSEZ Guillaume Examinateur Dr SERGEANT Nicolas Rapporteur Dr AGBULU Onnik Rapporteur Dr FURLING Denis Directeur de thèse EXPANSION DE TRIPLETS CTG ET ARRET PROLIFERATIF PRECOCE DES MYOBLASTES DM1 Thèse réalisée au sein du laboratoire : Thérapie des maladies du muscle strié - Institut de Myologie UPMC Univ. -
Burden of Rare Variants in ALS and Axonal Hereditary Neuropathy Genes Influence Survival In
International Journal of Molecular Sciences Article Burden of Rare Variants in ALS and Axonal Hereditary Neuropathy Genes Influence Survival in ALS: Insights from a Next Generation Sequencing Study of an Italian ALS Cohort Stefania Scarlino 1, Teuta Domi 1, Laura Pozzi 1 , Alessandro Romano 1, Giovanni Battista Pipitone 2, Yuri Matteo Falzone 1,3, Lorena Mosca 4, Silvana Penco 4, Christian Lunetta 5, Valeria Sansone 5,6, Lucio Tremolizzo 7, Raffaella Fazio 3, Federica Agosta 8, 3,8,9,10 2 1,3, , 1, Massimo Filippi , Paola Carrera , Nilo Riva * y and Angelo Quattrini y 1 Experimental Neuropathology Unit, Institute of Experimental Neurology (INSPE), Division of Neuroscience, San Raffaele Scientific Institute, 20132 Milan, Italy; [email protected] (S.S.); [email protected] (T.D.); [email protected] (L.P.); [email protected] (A.R.); [email protected] (Y.M.F.); [email protected] (A.Q.) 2 Laboratory of Clinical Molecular Biology, Unit of Genomics for Human Disease Diagnosis, Division of Genetics and Cell Biology, San Raffaele Scientific Institute, 20132 Milan, Italy; [email protected] (G.B.P.); [email protected] (P.C.) 3 Neurology Unit, San Raffaele Scientific Institute, 20132 Milan, Italy; fazio.raff[email protected] (R.F.); fi[email protected] (M.F.) 4 Medical Genetic Unit, Department of Laboratory Medicine, Niguarda Hospital, 20132 Milan, Italy; [email protected] (L.M.); [email protected] (S.P.) 5 NEuroMuscular Omnicentre (NEMO), Fondazione Serena Onlus, Milan 20132, Italy; [email protected] -
High Resolution Physical and Comparative Maps of Horse
HIGH RESOLUTION PHYSICAL AND COMPARATIVE MAPS OF HORSE CHROMOSOMES 14 (ECA14) AND 21 (ECA21) A Thesis by GLENDA GOH Submitted to the Office of Graduate Studies of Texas A&M University in partial fulfillment of the requirements for the degree of MASTER OF SCIENCE May 2005 Major Subject: Genetics HIGH RESOLUTION PHYSICAL AND COMPARATIVE MAPS OF HORSE CHROMOSOMES 14 (ECA14) AND 21 (ECA21) A Thesis by GLENDA GOH Submitted to Texas A&M University in partial fulfillment of the requirements for the degree of MASTER OF SCIENCE Approved as to style and content by: _________________________ _________________________ Bhanu P. Chowdhary Loren C. Skow (Chair of Committee) (Member) _________________________ _________________________ James N. Derr James E. Womack (Member) (Member) _________________________ _________________________ Geoffrey Kapler Evelyn Tiffany-Castiglioni (Chair of Genetics Faculty) (Head of Department) May 2005 Major Subject: Genetics iii ABSTRACT High Resolution Physical and Comparative Maps of Horse Chromosomes 14 (ECA14) and 21 (ECA21). (May 2005) Glenda Goh, B.S. (Hons.), Flinders University of South Australia Chair of Advisory Committee: Dr. Bhanu P. Chowdhary In order to identify genes or markers responsible for economically important traits in the horse, the development of high resolution gene maps of individual equine chromosomes is essential. We herein report the construction of high resolution physically ordered radiation hybrid (RH) and comparative maps for horse chromosomes 14 and 21 (ECA14 and ECA21). These chromosomes predominantly share correspondence with human chromosome 5 (HSA5), though a small region on the proximal part of ECA21 corresponds to a ~5Mb region from the short arm of HSA19. The map for ECA14 consists of 128 markers (83 Type I and 45 Type II) and spans a total of 1828cR.Compared to this, the map of ECA21 is made up of 90 markers (64 Type I and 26 Type II), that segregate into two linkage groups spanning 278 and 760cR each. -
Regulation of the Mouse Αb-Crystallin and MKBP/Hspb2 Promoter Activities by Shared and Gene Specific Intergenic Elements: the Importance of Context Dependency
Int. J. Dev. Biol. 51: 689-700 (2007) doi: 10.1387/ijdb.072302ss Original Article Regulation of the mouse αB-crystallin and MKBP/HspB2 promoter activities by shared and gene specific intergenic elements: the importance of context dependency SHIVALINGAPPA K. SWAMYNATHAN# and JORAM PIATIGORSKY* Laboratory of Molecular and Developmental Biology, National Eye Institute, NIH, Bethesda, Maryland, USA ABSTRACT The closely linked (863 bp), divergently arranged mouse myotonic dystrophy kinase binding protein (Mkbp)/HspB2 and small heat shock protein (shsp)/αB-crystallin genes have different patterns of tissue-specific expression. We showed previously that an intergenic enhancing region (-436/-257 relative to αB-crystallin transcription start site) selectively activates the αB- crystallin promoter in an orientation-dependent manner (Swamynathan, S.K. and J. Piatigorsky 2002. J. Biol. Chem. 277:49700-6). Here we show that cis-elements αBE1 (-420/-396) and αBE3 (-320/ -300) functionally interact with glucocorticoid receptor (GR) and Sp1, respectively, both in vitro and in vivo. αBE1:GR regulates both the HspB2 and αB-crystallin promoters, while αBE3:Sp1 selectively regulates the αB-crystallin promoter, as judged by mutagenesis and co-transfection tests. Enhancer blocking assays indicate that the -836/-622 fragment can act as a negative regulator in transfection tests, raising the possibility that it contributes to the differential expression of the proximal HspB2 promoter and distal αB-crystallin promoter. Finally, experi- ments utilizing transiently transfected cells and transgenic mice show that two conserved E-box elements (-726/-721 and -702/-697) bind nuclear proteins and differentially regulate the HspB2 and αB-crystallin promoters in a tissue-specific manner.