Development of Cortical Folding During Evolution and Ontogeny
Total Page:16
File Type:pdf, Size:1020Kb
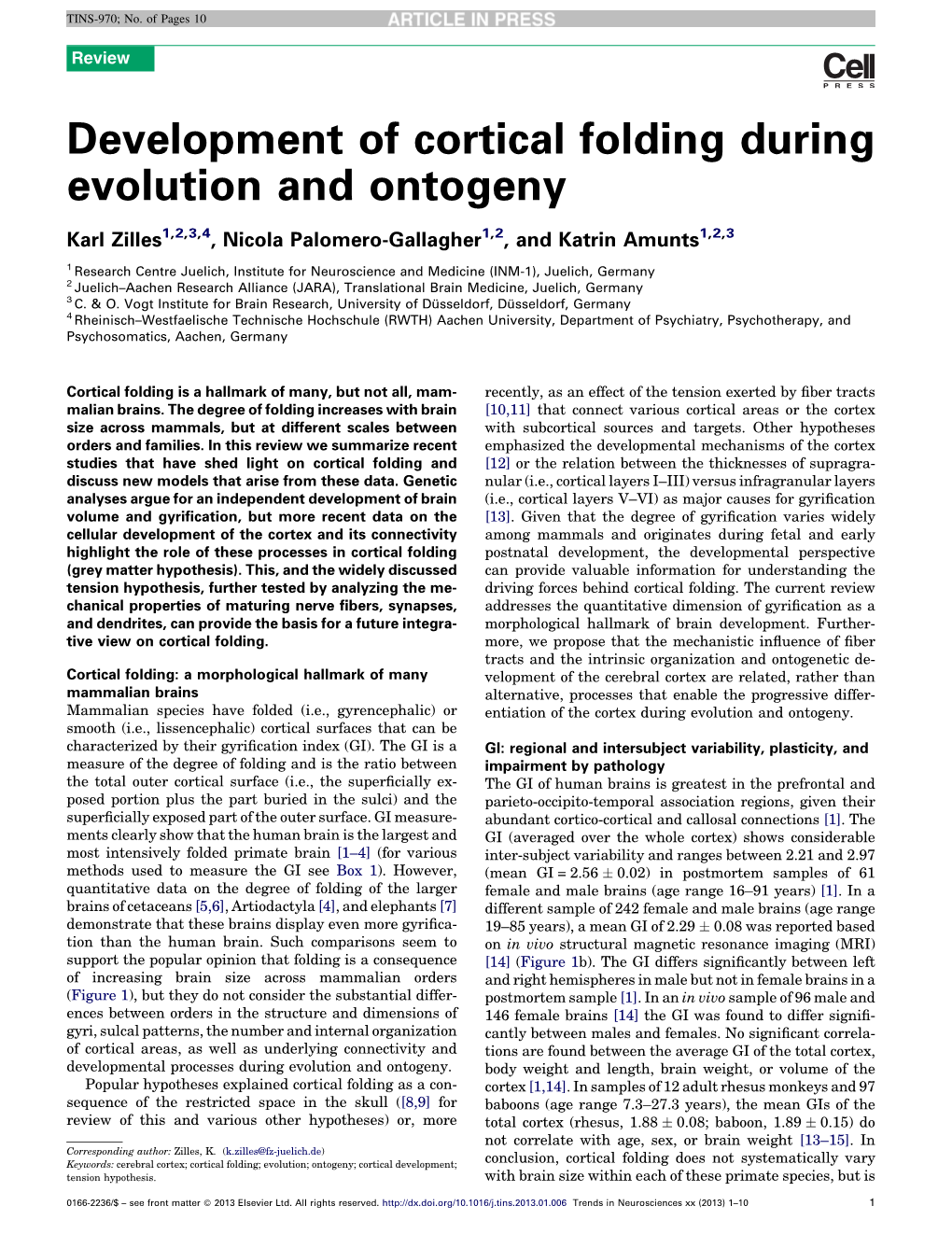
Load more
Recommended publications
-
Suppression of DNA Double-Strand Break Formation by DNA Polymerase B in Active DNA Demethylation Is Required for Development of Hippocampal Pyramidal Neurons
9012 • The Journal of Neuroscience, November 18, 2020 • 40(47):9012–9027 Development/Plasticity/Repair Suppression of DNA Double-Strand Break Formation by DNA Polymerase b in Active DNA Demethylation Is Required for Development of Hippocampal Pyramidal Neurons Akiko Uyeda,1 Kohei Onishi,1 Teruyoshi Hirayama,1,2,3 Satoko Hattori,4 Tsuyoshi Miyakawa,4 Takeshi Yagi,1,2 Nobuhiko Yamamoto,1 and Noriyuki Sugo1 1Graduate School of Frontier Biosciences, Osaka University, Suita, Osaka 565-0871, Japan, 2AMED-CREST, Japan Agency for Medical Research and Development, Suita, Osaka 565-0871, Japan, 3Department of Anatomy and Developmental Neurobiology, Tokushima University Graduate School of Medical Sciences, Kuramoto, Tokushima 770-8503, Japan, and 4Institute for Comprehensive Medical Science, Fujita Health University, Toyoake, Aichi 470-1192, Japan Genome stability is essential for brain development and function, as de novo mutations during neuronal development cause psychiatric disorders. However, the contribution of DNA repair to genome stability in neurons remains elusive. Here, we demonstrate that the base excision repair protein DNA polymerase b (Polb) is involved in hippocampal pyramidal neuron fl/fl differentiation via a TET-mediated active DNA demethylation during early postnatal stages using Nex-Cre/Polb mice of ei- ther sex, in which forebrain postmitotic excitatory neurons lack Polb expression. Polb deficiency induced extensive DNA dou- ble-strand breaks (DSBs) in hippocampal pyramidal neurons, but not dentate gyrus granule cells, and to a lesser extent in neocortical neurons, during a period in which decreased levels of 5-methylcytosine and 5-hydroxymethylcytosine were observed in genomic DNA. Inhibition of the hydroxylation of 5-methylcytosine by expression of microRNAs miR-29a/b-1 diminished DSB formation. -
Gyrification from Constrained Cortical Expansion
Gyrification from constrained cortical expansion Tuomas Tallinena, Jun Young Chungb, John S. Bigginsc, and L. Mahadevand,e,f,1 aDepartment of Physics and Nanoscience Center, University of Jyväskylä, FI-40014 Jyväskylä, Finland; bSchool of Engineering and Applied Sciences, Harvard University, Cambridge, MA 02138; cCavendish Laboratory, Cambridge University, Cambridge CB3 0HE, United Kingdom; dWyss Institute for Biologically Inspired Engineering, eKavli Institute for Bionano Science and Technology, and fSchool of Engineering and Applied Sciences, Department of Organismic and Evolutionary Biology, and Department of Physics, Harvard University, Cambridge, MA 02138 Edited* by John W. Hutchinson, Harvard University, Cambridge, MA, and approved July 15, 2014 (received for review April 1, 2014) The exterior of the mammalian brain—the cerebral cortex—has from an unregulated and unpatterned growth of the cortex rel- a conserved layered structure whose thickness varies little across ative to sublayers. species. However, selection pressures over evolutionary time scales Nevertheless, there is as yet no explicit biologically and physi- have led to cortices that have a large surface area to volume ratio in cally plausible model that can convincingly reproduce individual some organisms, with the result that the brain is strongly convo- sulci and gyri, let alone the complex patterns of sulci and gyri luted into sulci and gyri. Here we show that the gyrification can found in the brain. Early attempts to mechanically model brain arise as a nonlinear consequence of a simple mechanical instability folding (13) were rooted in the physics of wrinkling and assumed driven by tangential expansion of the gray matter constrained by a thin stiff layer of gray matter that grows relative to a thick soft the white matter. -
Congenital Microcephaly
View metadata, citation and similar papers at core.ac.uk brought to you by CORE provided by Sussex Research Online American Journal of Medical Genetics Part C (Seminars in Medical Genetics) ARTICLE Congenital Microcephaly DIANA ALCANTARA AND MARK O'DRISCOLL* The underlying etiologies of genetic congenital microcephaly are complex and multifactorial. Recently, with the exponential growth in the identification and characterization of novel genetic causes of congenital microcephaly, there has been a consolidation and emergence of certain themes concerning underlying pathomechanisms. These include abnormal mitotic microtubule spindle structure, numerical and structural abnormalities of the centrosome, altered cilia function, impaired DNA repair, DNA Damage Response signaling and DNA replication, along with attenuated cell cycle checkpoint proficiency. Many of these processes are highly interconnected. Interestingly, a defect in a gene whose encoded protein has a canonical function in one of these processes can often have multiple impacts at the cellular level involving several of these pathways. Here, we overview the key pathomechanistic themes underlying profound congenital microcephaly, and emphasize their interconnected nature. © 2014 Wiley Periodicals, Inc. KEY WORDS: cell division; mitosis; DNA replication; cilia How to cite this article: Alcantara D, O'Driscoll M. 2014. Congenital microcephaly. Am J Med Genet Part C Semin Med Genet 9999:1–16. INTRODUCTION mid‐gestation although glial cell division formation of the various cortical layers. and consequent brain volume enlarge- Furthermore, differentiating and devel- Congenital microcephaly, an occipital‐ ment does continue after birth [Spalding oping neurons must migrate to their frontal circumference of equal to or less et al., 2005]. Impaired neurogenesis is defined locations to construct the com- than 2–3 standard deviations below the therefore most obviously reflected clini- plex architecture and laminar layered age‐related population mean, denotes cally as congenital microcephaly. -
NERVOUS SYSTEM هذا الملف لالستزادة واثراء المعلومات Neuropsychiatry Block
NERVOUS SYSTEM هذا الملف لﻻستزادة واثراء المعلومات Neuropsychiatry block. قال تعالى: ) َو َل َق د َخ َل قنَا ا ِْلن َسا َن ِمن ُس ََل َل ة ِ من ِطي ن }12{ ثُ م َجعَ لنَاه ُ نُ ط َفة فِي َق َرا ر م ِكي ن }13{ ثُ م َخ َل قنَا ال ُّن ط َفة َ َع َل َقة َف َخ َل قنَا ا لعَ َل َقة َ ُم ضغَة َف َخ َل قنَا ا ل ُم ضغَة َ ِع َظا ما َف َك َس ونَا ا ل ِع َظا َم َل ح ما ثُ م أَن َشأنَاه ُ َخ ل قا آ َخ َر َفتَبَا َر َك ّللا ُ أَ ح َس ُن ا ل َخا ِل ِقي َن }14{( Resources BRS Embryology Book. Pathoma Book ( IN DEVELOPMENTAL ANOMALIES PART ). [email protected] 1 OVERVIEW A- Central nervous system (CNS) is formed in week 3 of development, during which time the neural plate develops. The neural plate, consisting of neuroectoderm, becomes the neural tube, which gives rise to the brain and spinal cord. B- Peripheral nervous system (PNS) is derived from three sources: 1. Neural crest cells 2. Neural tube, which gives rise to all preganglionic autonomic nerves (sympathetic and parasympathetic) and all nerves (-motoneurons and -motoneurons) that innervate skeletal muscles 3. Mesoderm, which gives rise to the dura mater and to connective tissue investments of peripheral nerve fibers (endoneurium, perineurium, and epineurium) DEVELOPMENT OF THE NEURAL TUBE Neurulation refers to the formation and closure of the neural tube. BMP-4 (bone morphogenetic protein), noggin (an inductor protein), chordin (an inductor protein), FGF-8 (fibroblast growth factor), and N-CAM (neural cell adhesion molecule) appear to play a role in neurulation. -
The Hominoid-Specific Gene TBC1D3 Promotes Generation of Basal Neural
1 The hominoid-specific gene TBC1D3 promotes generation of basal neural 2 progenitors and induces cortical folding in mice 3 4 Xiang-Chun Ju1,3,9, Qiong-Qiong Hou1,3,9, Ai-Li Sheng1, Kong-Yan Wu1, Yang Zhou8, 5 Ying Jin8, Tieqiao Wen7, Zhengang Yang6, Xiaoqun Wang2,5, Zhen-Ge Luo1,2,3,4 6 7 1Institute of Neuroscience, State Key Laboratory of Neuroscience, Shanghai Institutes for 8 Biological Sciences, Chinese Academy of Sciences, Shanghai, China. 9 2CAS Center for Excellence in Brain Science and Intelligence Technology, Shanghai, 10 China. 11 3Chinese Academy of Sciences University, Beijing, China. 12 4ShanghaiTech University, Shanghai, China 13 5Institute of Biophysics, Chinese Academy of Sciences, Beijing, China. 14 6Institutes of Brain Science, State Key Laboratory of Medical Neurobiology, Fudan 15 University, Shanghai, China. 16 7School of Life Sciences, Shanghai University, Shanghai, China. 17 8The Institute of Health Sciences, Shanghai Institutes for Biological Sciences, Chinese 18 Academy of Sciences, Shanghai, China. 19 9Co-first author 20 Correspondence should be addressed to Z.G.L ([email protected]) 21 22 Author contributions 23 X.-C.J and Q.-Q. H performed most experiments, analyzed data and wrote the paper. 24 A.-L.S helped with in situ hybridization. K.-Y.W assisted with imaging analysis. T.W 25 helped with the construction of nestin promoter construct. Y. Z and Y. J helped with 26 ReNeuron cell culture and analysis. Z.Y provided human fetal samples and assisted with 1 27 immunohistochemistry analysis. X.W provided help with live-imaging analysis. Z.-G.L 28 supervised the whole study, designed the research, analyzed data and wrote the paper. -
Anatomically Distinct Patterns of Diffusion MRI Coherence
NeuroImage 79 (2013) 412–422 Contents lists available at SciVerse ScienceDirect NeuroImage journal homepage: www.elsevier.com/locate/ynimg Radial and tangential neuronal migration pathways in the human fetal brain: Anatomically distinct patterns of diffusion MRI coherence James Kolasinski a,c,1, Emi Takahashi a,b,⁎,1, Allison A. Stevens a, Thomas Benner a, Bruce Fischl a, Lilla Zöllei a,b,2, P. Ellen Grant a,b,2 a Athinoula A. Martinos Center for Biomedical Imaging, Massachusetts General Hospital, Harvard Medical School, Charlestown, MA 02119, USA b Division of Newborn Medicine, Department of Medicine/Fetal–Neonatal Neuroimaging and Developmental Science Center, Children's Hospital Boston, Harvard Medical School, Boston, MA 02115, USA c Centre for Functional Magnetic Resonance Imaging of the Brain (FMRIB), Nuffield Department of Clinical Neurosciences, University of Oxford, Oxford OX3 9DU, UK article info abstract Article history: Corticogenesis is underpinned by a complex process of subcortical neuroproliferation, followed by highly orches- Accepted 29 April 2013 trated cellular migration. A greater appreciation of the processes involved in human fetal corticogenesis is vital to Available online 11 May 2013 gaining an understanding of how developmental disturbances originating in gestation could establish a variety of complex neuropathology manifesting in childhood, or even in adult life. Magnetic resonance imaging modalities offer a unique insight into anatomical structure, and increasingly infer information regarding underlying microstructure in the human brain. In this study we applied a combination of high-resolution structural and diffusion-weighted magnetic resonance imaging to a unique cohort of three post-mortem fetal brain specimens, aged between 19 and 22 post-conceptual weeks. -
Heterogeneity in Ventricular Zone Neural Precursors Contributes to Neuronal Fate Diversity in the Postnatal Neocortex
7028 • The Journal of Neuroscience, May 19, 2010 • 30(20):7028–7036 Development/Plasticity/Repair Heterogeneity in Ventricular Zone Neural Precursors Contributes to Neuronal Fate Diversity in the Postnatal Neocortex Elizabeth K. Stancik,1 Ivan Navarro-Quiroga,1 Robert Sellke,2 and Tarik F. Haydar1 1Center for Neuroscience Research, Children’s National Medical Center, Washington, DC 20010, and 2University of Maryland School of Medicine, Baltimore, Maryland 21201 The recent discovery of short neural precursors (SNPs) in the murine neocortical ventricular zone (VZ) challenges the widely held view that radial glial cells (RGCs) are the sole occupants of this germinal compartment and suggests that precursor variety is an important factor of brain development. Here, we use in utero electroporation and genetic fate mapping to show that SNPs and RGCs cohabit the VZ but display different cell cycle kinetics and generate phenotypically different progeny. In addition, we find that RGC progeny undergo additional rounds of cell division as intermediate progenitor cells (IPCs), whereas SNP progeny generally produce postmitotic neurons directly from the VZ. By clearly defining SNPs as bona fide VZ residents, separate from both RGCs and IPCs, and uncovering their unique proliferativeandlineageproperties,theseresultsdemonstratehowindividualneuralprecursorgroupsintheembryonicrodentVZcreate diversity in the overlying neocortex. Introduction progenitors properly form the cerebral cortex as well as for elu- The ventricular zone (VZ) of the dorsal telencephalon contains cidating possible mechanisms of species-specific diversity. the progenitor cells that produce all of the various excitatory In addition to the neural precursors in the VZ, a separate class neurons of the mature neocortex. This process occurs during of neuronal progenitors has been described in the overlying sub- prenatal mammalian brain development through a precisely reg- ventricular zone (SVZ). -
Development and Evolution of the Human Neocortex
Leading Edge Review Development and Evolution of the Human Neocortex Jan H. Lui,1,2,3 David V. Hansen,1,2,4 and Arnold R. Kriegstein1,2,* 1Eli and Edythe Broad Center of Regeneration Medicine and Stem Cell Research, University of California, San Francisco, 35 Medical Center Way, San Francisco, CA 94143, USA 2Department of Neurology 3Biomedical Sciences Graduate Program University of California, San Francisco, 513 Parnassus Avenue, San Francisco, CA 94143, USA 4Present address: Department of Neuroscience, Genentech, Inc., 1 DNA Way, MS 230B, South San Francisco, CA 94080, USA *Correspondence: [email protected] DOI 10.1016/j.cell.2011.06.030 The size and surface area of the mammalian brain are thought to be critical determinants of intel- lectual ability. Recent studies show that development of the gyrated human neocortex involves a lineage of neural stem and transit-amplifying cells that forms the outer subventricular zone (OSVZ), a proliferative region outside the ventricular epithelium. We discuss how proliferation of cells within the OSVZ expands the neocortex by increasing neuron number and modifying the trajectory of migrating neurons. Relating these features to other mammalian species and known molecular regulators of the mouse neocortex suggests how this developmental process could have emerged in evolution. Introduction marsupials begin to reveal how differences in neural progenitor Evolution of the neocortex in mammals is considered to be a key cell populations can result in neocortices of variable size and advance that enabled higher cognitive function. However, neo- shape. Increases in neocortical volume and surface area, partic- cortices of different mammalian species vary widely in shape, ularly in the human, are related to the expansion of progenitor size, and neuron number (reviewed by Herculano-Houzel, 2009). -
From Genes to Folds: a Review of Cortical Gyrification Theory
View metadata, citation and similar papers at core.ac.uk brought to you by CORE provided by Springer - Publisher Connector Brain Struct Funct (2015) 220:2475–2483 DOI 10.1007/s00429-014-0961-z REVIEW From genes to folds: a review of cortical gyrification theory Lisa Ronan • Paul C. Fletcher Received: 5 September 2014 / Accepted: 6 December 2014 / Published online: 16 December 2014 Ó The Author(s) 2014. This article is published with open access at Springerlink.com Abstract Cortical gyrification is not a random process. Introduction: key characteristics of gyrification Instead, the folds that develop are synonymous with the functional organization of the cortex, and form patterns A wing would be a most mystifying structure if one that are remarkably consistent across individuals and even did not know that birds flew. One might observe that some species. How this happens is not well understood. it could be extended a considerable distance that it Although many developmental features and evolutionary had a smooth covering of feathers with conspicuous adaptations have been proposed as the primary cause of markings, that it was operated by powerful muscles, gyrencephaly, it is not evident that gyrification is reducible and that strength and lightness were prominent fea- in this way. In recent years, we have greatly increased our tures of its construction. These are important facts, understanding of the multiple factors that influence cortical but by themselves they do not tell us that birds fly. folding, from the action of genes in health and disease to Yet without knowing this, and without understanding evolutionary adaptations that characterize distinctions something of the principles of flight, a more detailed between gyrencephalic and lissencephalic cortices. -
Morphometric Analysis of Brain in Newborn with Congenital Diaphragmatic Hernia
brain sciences Article Morphometric Analysis of Brain in Newborn with Congenital Diaphragmatic Hernia Martina Lucignani 1, Daniela Longo 2, Elena Fontana 2, Maria Camilla Rossi-Espagnet 2,3, Giulia Lucignani 2, Sara Savelli 4, Stefano Bascetta 4, Stefania Sgrò 5, Francesco Morini 6, Paola Giliberti 6 and Antonio Napolitano 1,* 1 Medical Physics Department, Bambino Gesù Children’s Hospital, IRCCS, 00165 Rome, Italy; [email protected] 2 Neuroradiology Unit, Imaging Department, Bambino Gesù Children’s Hospital, IRCCS, 00165 Rome, Italy; [email protected] (D.L.); [email protected] (E.F.); [email protected] (M.C.R.-E.); [email protected] (G.L.) 3 NESMOS Department, Sant’Andrea Hospital, Sapienza University, 00189 Rome, Italy 4 Imaging Department, Bambino Gesù Children’s Hospital and Research Institute, 00165 Rome, Italy; [email protected] (S.S.); [email protected] (S.B.) 5 Department of Anesthesia and Critical Care, Bambino Gesù Children’s Hospital, IRCCS, 00165 Rome, Italy; [email protected] 6 Department of Medical and Surgical Neonatology, Bambino Gesù Children’s Hospital, IRCCS, 00165 Rome, Italy; [email protected] (F.M.); [email protected] (P.G.) * Correspondence: [email protected]; Tel.: +39-333-3214614 Abstract: Congenital diaphragmatic hernia (CDH) is a severe pediatric disorder with herniation of abdominal viscera into the thoracic cavity. Since neurodevelopmental impairment constitutes a common outcome, we performed morphometric magnetic resonance imaging (MRI) analysis on Citation: Lucignani, M.; Longo, D.; CDH infants to investigate cortical parameters such as cortical thickness (CT) and local gyrification Fontana, E.; Rossi-Espagnet, M.C.; index (LGI). -
BMP Signaling Suppresses Gemc1 Expression and Ependymal
www.nature.com/scientificreports OPEN BMP signaling suppresses Gemc1 expression and ependymal diferentiation of mouse telencephalic progenitors Hanae Omiya1, Shima Yamaguchi1, Tomoyuki Watanabe1, Takaaki Kuniya1, Yujin Harada1, Daichi Kawaguchi1* & Yukiko Gotoh1,2* The lateral ventricles of the adult mammalian brain are lined by a single layer of multiciliated ependymal cells, which generate a fow of cerebrospinal fuid through directional beating of their cilia as well as regulate neurogenesis through interaction with adult neural stem cells. Ependymal cells are derived from a subset of embryonic neural stem-progenitor cells (NPCs, also known as radial glial cells) that becomes postmitotic during the late embryonic stage of development. Members of the Geminin family of transcriptional regulators including GemC1 and Mcidas play key roles in the diferentiation of ependymal cells, but it remains largely unclear what extracellular signals regulate these factors and ependymal diferentiation during embryonic and early-postnatal development. We now show that the levels of Smad1/5/8 phosphorylation and Id1/4 protein expression—both of which are downstream events of bone morphogenetic protein (BMP) signaling—decline in cells of the ventricular- subventricular zone in the mouse lateral ganglionic eminence in association with ependymal diferentiation. Exposure of postnatal NPC cultures to BMP ligands or to a BMP receptor inhibitor suppressed and promoted the emergence of multiciliated ependymal cells, respectively. Moreover, treatment of embryonic NPC cultures with BMP ligands reduced the expression level of the ependymal marker Foxj1 and suppressed the emergence of ependymal-like cells. Finally, BMP ligands reduced the expression levels of Gemc1 and Mcidas in postnatal NPC cultures, whereas the BMP receptor inhibitor increased them. -
Early Dorsomedial Tissue Interactions Regulate Gyrification of Distal
ARTICLE https://doi.org/10.1038/s41467-019-12913-z OPEN Early dorsomedial tissue interactions regulate gyrification of distal neocortex Victor V. Chizhikov1*, Igor Y. Iskusnykh 1, Ekaterina Y. Steshina1, Nikolai Fattakhov 1, Anne G. Lindgren2, Ashwin S. Shetty3, Achira Roy 4, Shubha Tole3 & Kathleen J. Millen4,5* The extent of neocortical gyrification is an important determinant of a species’ cognitive abilities, yet the mechanisms regulating cortical gyrification are poorly understood. We 1234567890():,; uncover long-range regulation of this process originating at the telencephalic dorsal midline, where levels of secreted Bmps are maintained by factors in both the neuroepithelium and the overlying mesenchyme. In the mouse, the combined loss of transcription factors Lmx1a and Lmx1b, selectively expressed in the midline neuroepithelium and the mesenchyme respec- tively, causes dorsal midline Bmp signaling to drop at early neural tube stages. This alters the spatial and temporal Wnt signaling profile of the dorsal midline cortical hem, which in turn causes gyrification of the distal neocortex. Our study uncovers early mesenchymal- neuroepithelial interactions that have long-range effects on neocortical gyrification and shows that lissencephaly in mice is actively maintained via redundant genetic regulation of dorsal midline development and signaling. 1 Department of Anatomy and Neurobiology, University of Tennessee Health Science Center, Memphis, TN 38163, USA. 2 Department of Human Genetics, University of Chicago, Chicago, IL 60637, USA. 3 Department of Biological Sciences, Tata Institute of Fundamental Research, Mumbai, India. 4 Center for Integrative Brain Research, Seattle Children’s Research Institute, Seattle, WA 98101, USA. 5 Department of Pediatrics, University of Washington, Seattle, WA 98101, USA.