DNA Sequence Context Controls the Binding and Processivity of the T7 DNA Primase
Total Page:16
File Type:pdf, Size:1020Kb
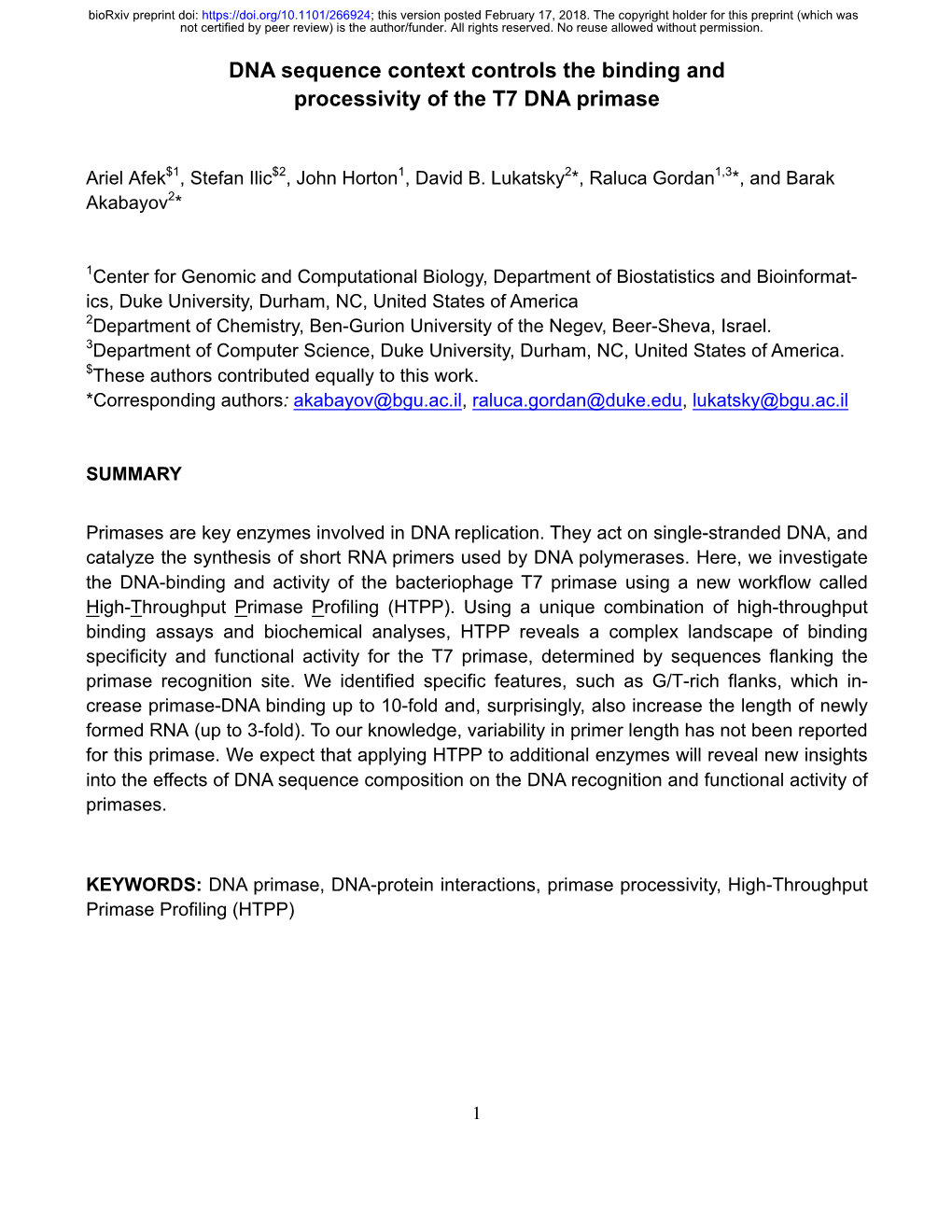
Load more
Recommended publications
-
Processivity of DNA Polymerases: Two Mechanisms, One Goal Zvi Kelman1*, Jerard Hurwitz1 and Mike O’Donnell2
Minireview 121 Processivity of DNA polymerases: two mechanisms, one goal Zvi Kelman1*, Jerard Hurwitz1 and Mike O’Donnell2 Replicative DNA polymerases are highly processive Processive DNA synthesis by cellular replicases and the enzymes that polymerize thousands of nucleotides without bacteriophage T4 replicase dissociating from the DNA template. The recently Until recently, the only mechanism for high processivity determined structure of the Escherichia coli bacteriophage that was understood in detail was that utilized by cellular T7 DNA polymerase suggests a unique mechanism that replicases and the replicase of bacteriophage T4. This underlies processivity, and this mechanism may generalize mechanism involves a ring-shaped protein called a ‘DNA to other replicative polymerases. sliding clamp’ that encircles the DNA and tethers the polymerase catalytic unit to the DNA [3,4]. The three- Addresses: 1Department of Molecular Biology, Memorial Sloan- dimensional structures of several sliding clamps have been Kettering Cancer Center, 1275 York Avenue, New York, NY 10021, 2 determined: the eukaryotic proliferating cell nuclear USA and Laboratory of DNA Replication, Howard Hughes Medical β Institute, The Rockefeller University, 1230 York Avenue, New York, NY antigen (PCNA) [5,6]; the subunit of the prokaryotic 10021, USA. DNA polymerase III [7]; and the bacteriophage T4 gene 45 protein (gp45) (J Kuriyan, personal communication) *Corresponding author. (Figure 1). The overall structure of these clamps is very E-mail: [email protected] similar; the PCNA, β subunit and gp45 rings are super- Structure 15 February 1998, 6:121–125 imposable [8]. Each ring has similar dimensions and a http://biomednet.com/elecref/0969212600600121 central cavity large enough to accommodate duplex DNA (Figure 1). -
Synergy of Topoisomerase and Structural-Maintenance
Synergy of topoisomerase and structural-maintenance- of-chromosomes proteins creates a universal pathway to simplify genome topology Enzo Orlandinia, Davide Marenduzzob, and Davide Michielettob,1 aDipartimento di Fisica e Astronomia “Galileo Galilei,” Sezione Istituto Nazionale di Fisica Nucleare, Universita` degli Studi di Padova, I-35131 Padova, Italy; and bSchool of Physics and Astronomy, University of Edinburgh, Edinburgh EH9 3FD, United Kingdom Edited by Michael L. Klein, Institute of Computational Molecular Science, Temple University, Philadelphia, PA, and approved March 14, 2019 (received for review September 6, 2018) Topological entanglements severely interfere with important bio- cal simplification. Our simulations reveal that this mechanism is logical processes. For this reason, genomes must be kept unknot- independent of either substrate condensation or crowding and is ted and unlinked during most of a cell cycle. Type II topoisomerase therefore likely to lead to unknotting and unlinking even under (TopoII) enzymes play an important role in this process but the extreme conditions such as those in the cell nucleus. Finally, we precise mechanisms yielding systematic disentanglement of DNA discuss our model in the context of recent experiments report- in vivo are not clear. Here we report computational evidence that ing that SMC proteins are essential to achieve correct sister structural-maintenance-of-chromosomes (SMC) proteins—such as chromatid decatenation in metaphase (23), that DNA damage cohesins and condensins—can cooperate with TopoII to establish is frequently found in front of cohesin motion (24), and that a synergistic mechanism to resolve topological entanglements. there is a remarkable low frequency of knots in intracellular SMC-driven loop extrusion (or diffusion) induces the spatial local- chromatin (17). -
Herpes Simplex Virus 1 Activates Cdc2 to Recruit Topoisomerase II for Post-DNA Synthesis Expression of Late Genes
Herpes simplex virus 1 activates cdc2 to recruit topoisomerase II␣ for post-DNA synthesis expression of late genes Sunil J. Advani*†, Ralph R. Weichselbaum†, and Bernard Roizman*‡ *The Marjorie B. Kovler Viral Oncology Laboratories and †Department of Radiation and Cellular Oncology, 910 East 58th Street, University of Chicago, Chicago, IL 60637 Contributed by Bernard Roizman, February 6, 2003 A subset (␥2) of late herpes simplex virus 1 genes depends on viral acquire a new, viral partner to compensate for the loss of cyclin DNA synthesis for its expression. For optimal expression, a small B revealed that cdc2 interacted physically and functionally with number of these genes, exemplified by US11, also requires two the viral DNA polymerase processivity factor encoded by the ␣ viral proteins, the protein infected cell protein (ICP) 22 and UL42 ORF (7). Taken together, these studies indicated that the protein kinase UL13. Earlier we showed that UL13 and ICP22 activated cdc2 played a role in late viral gene expression but left mediate the stabilization of cdc2 and the replacement of its cellular unanswered the question of the role of the cdc2–UL42 complex partner, cyclin B, with the viral DNA polymerase processivity factor in this process. U 42. Here we report that cdc2 and its new partner, U 42, bind a L L In the search for a potential target of the cdc2–UL42 complex, phosphorylated form of topoisomerase II␣. The posttranslational we took cognizance that in uninfected cells topoisomerase II␣ is modification of topoisomerase II␣ and its interaction with cdc2– modified in a cell cycle-dependent manner. -
Autonomous Replication of the Conjugative Transposon Tn916
Wright and Grossman 1 1 2 Autonomous replication of the conjugative transposon Tn916 3 4 Laurel D. Wright and Alan D. Grossman* 5 Department of Biology 6 Massachusetts Institute of Technology 7 Cambridge, MA 02139 8 9 10 Short title: Autonomous replication of Tn916 11 12 13 *Corresponding author: 14 Department of Biology 15 Building 68-530 16 Massachusetts Institute of Technology 17 Cambridge, MA 02139 18 phone: 617-253-1515 19 email: [email protected] 20 21 22 23 24 25 Wright and Grossman 2 26 Abstract 27 Integrative and conjugative elements (ICEs), also known as conjugative transposons, are self- 28 transferable elements that are widely distributed among bacterial phyla and are important drivers 29 of horizontal gene transfer. Many ICEs carry genes that confer antibiotic resistances to their host 30 cells and are involved in the dissemination of these resistance genes. ICEs reside in host 31 chromosomes, but under certain conditions can excise to form a plasmid that is typically the 32 substrate for transfer. A few ICEs are known to undergo autonomous replication following 33 activation. However, it is not clear if autonomous replication is a general property of many 34 ICEs. We found that Tn916, the first conjugative transposon identified, replicates autonomously 35 via a rolling circle mechanism. Replication of Tn916 was dependent on the relaxase encoded by 36 orf20 of Tn916. The origin of transfer of Tn916, oriT(916), also functioned as an origin of 37 replication. Using immunoprecipitation and mass spectrometry, we found that the relaxase 38 (Orf20) and the two putative helicase processivity factors (Orf22 and Orf23) encoded by Tn916 39 likely interact in a complex and that the Tn916 relaxase contains a previously unidentified 40 conserved helix-turn-helix domain in its N-terminal region that is required for relaxase function 41 and replication. -
Switch-Like Control of Helicase Processivity by Single-Stranded DNA
bioRxiv preprint doi: https://doi.org/10.1101/2020.07.09.194779; this version posted July 9, 2020. The copyright holder for this preprint (which was not certified by peer review) is the author/funder, who has granted bioRxiv a license to display the preprint in perpetuity. It is made available under aCC-BY 4.0 International license. 1 Switch-like control of helicase processivity by single-stranded DNA 2 binding protein 3 4 Barbara Stekas1, Masayoshi Honda2, Maria Spies2, Yann R. Chemla1,3,* 5 1Department of Physics, University of Illinois, Urbana-Champaign, Urbana 6 2Department of Biochemistry, Carver College of Medicine, University of Iowa, Iowa City 7 3Center for the Physics of Living Cells, University of Illinois, Urbana-Champaign, Urbana 8 9 Helicases utilize the energy of NTP hydrolysis to translocate along single-stranded nucleic acids (NA) and 10 unwind the duplex. In the cell, helicases function in the context of other NA-associated proteins which 11 regulate helicase function. For example, single-stranded DNA binding proteins are known to enhance 12 helicase activity, although the underlying mechanisms remain largely unknown. F. acidarmanus XPD 13 helicase serves as a model for understanding the molecular mechanisms of Superfamily 2B helicases, and 14 previous work has shown that its activity is enhanced by the cognate single-stranded DNA binding protein 15 RPA2. Here, single-molecule optical trap measurements of the unwinding activity of a single XPD helicase 16 in the presence of RPA2 reveal a mechanism in which XPD interconverts between two states with different 17 processivities and transient RPA2 interactions stabilize the more processive state, activating a latent 18 “processivity switch” in XPD. -
The Thioredoxin Binding Domain of Bacteriophage T7 DNA
Proc. Natl. Acad. Sci. USA Vol. 94, pp. 479–484, January 1997 Biochemistry The thioredoxin binding domain of bacteriophage T7 DNA polymerase confers processivity on Escherichia coli DNA polymerase I (DNA replicationyT7 gene 5 proteinyKlenow DNA polymerase) ELLA BEDFORD,STANLEY TABOR, AND CHARLES C. RICHARDSON Department of Biological Chemistry and Molecular Pharmacology, Harvard Medical School, Boston, MA 02115 Contributed by Charles C. Richardson, November 14, 1996 ABSTRACT Bacteriophage T7 DNA polymerase shares 20 nt (8). In contrast to T7 DNA polymerase, it does not extensive sequence homology with Escherichia coli DNA poly- associate with any known accessory proteins. The three- merase I. However, in vivo, E. coli DNA polymerase I is dimensional structure of the large fragment of E. coli DNA involved primarily in the repair of DNA whereas T7 DNA polymerase I (Klenow DNA polymerase) is known (9, 10). The polymerase is responsible for the replication of the viral polymerase active site and DNA binding domain are in the genome. In accord with these roles, T7 DNA polymerase is carboxyl-terminal half of the molecule, and the 39 to 59 highly processive while E. coli DNA polymerase I has low proofreading exonuclease activity is located in a separate processivity. The high processivity of T7 DNA polymerase is domain at the amino-terminal half. Comparison of the se- achieved through tight binding to its processivity factor, E. coli quence of T7 DNA polymerase to E. coli DNA polymerase I thioredoxin. We have identified a unique 76-residue domain in reveals a 76-amino acid residue segment in T7 DNA polymer- T7 DNA polymerase responsible for this interaction. -
A Specific Subdomain in 29 DNA Polymerase Confers Both
A specific subdomain in 29 DNA polymerase confers both processivity and strand-displacement capacity Irene Rodrı´guez*,Jose´ M. La´ zaro*, Luis Blanco*, Satwik Kamtekar†, Andrea J. Berman†, Jimin Wang†, Thomas A. Steitz†‡§, Margarita Salas*¶, and Miguel de Vega* *Instituto de Biologı´aMolecular ‘‘Eladio Vin˜uela,’’ Consejo Superior de Investigaciones Cientı´ficas,Centro de Biologı´aMolecular ‘‘Severo Ochoa,’’ Universidad Auto´noma de Madrid, Canto Blanco, 28049 Madrid, Spain; and Departments of †Molecular Biophysics and Biochemistry and ‡Chemistry and §Howard Hughes Medical Institute, Yale University, New Haven, CT 06520 Edited by Charles C. Richardson, Harvard Medical School, Boston, MA, and approved March 24, 2005 (received for review January 24, 2005) Recent crystallographic studies of 29 DNA polymerase have replication at the origins located at both ends of the linear provided structural insights into its strand displacement and pro- genome by catalyzing the addition of the initial dAMP onto the cessivity. A specific insertion named terminal protein region 2 hydroxyl group of Ser-232 of the bacteriophage terminal protein (TPR2), present only in protein-primed DNA polymerases, together (TP), which acts as primer (reviewed in refs. 8–10). After a with the exonuclease, thumb, and palm subdomains, forms two transition stage in which a sequential switch from TP priming to tori capable of interacting with DNA. To analyze the functional role DNA priming occurs, the same polymerase molecule replicates of this insertion, we constructed a 29 DNA polymerase deletion the entire genome processively without dissociating from the mutant lacking TPR2 amino acid residues Asp-398 to Glu-420. DNA (11). Second, unlike 29 DNA polymerase, most repli- Biochemical analysis of the mutant DNA polymerase indicates that cases rely on accessory proteins to clamp the enzyme to the its DNA-binding capacity is diminished, drastically decreasing its DNA. -
Single-Molecule Analysis Reveals That the Lagging Strand Increases Replisome Processivity but Slows Replication Fork Progression
Single-molecule analysis reveals that the lagging strand increases replisome processivity but slows replication fork progression Nina Y. Yao1, Roxana E. Georgescu1, Jeff Finkelstein, and Michael E. O’Donnell2 Howard Hughes Medical Institute, Rockefeller University, 1230 York Avenue, New York, NY 10021 Contributed by Michael E. O’Donnell, June 3, 2009 (sent for review May 14, 2009) Single-molecule techniques are developed to examine mechanistic The current report examines the rate and processivity of the features of individual E. coli replisomes during synthesis of long E. coli replisome. The E. coli replisome consists of the ring DNA molecules. We find that single replisomes exhibit constant shaped hexameric DnaB helicase, DnaG primase, the DNA rates of fork movement, but the rates of different replisomes vary polymerase III* replicase (Pol III*), and  sliding clamps. over a surprisingly wide range. Interestingly, lagging strand syn- Processivity estimates of the E. coli replisome from ensemble thesis decreases the rate of the leading strand, suggesting that studies indicate a lower limit of 50 kb, and an average bulk rate lagging strand operations exert a drag on replication fork progres- of Ϸ500–700 nt/s at 37 °C (6, 7). Processivity measurements from sion. The opposite is true for processivity. The lagging strand other recent single-molecule studies differ over a wide range, significantly increases the processivity of the replisome, possibly from3kbtoϾ80 kb (8, 9). In each of these previous studies reflecting the increased grip to DNA provided by 2 DNA poly- replication was performed in the presence of excess proteins that merases anchored to sliding clamps on both the leading and could replace a replisome protein that dissociates from DNA, lagging strands. -
A Conserved Helicase Processivity Factor Is Needed for Conjugation and Replication of an Integrative and Conjugative Element
A conserved helicase processivity factor is needed for conjugation and replication of an integrative and conjugative element The MIT Faculty has made this article openly available. Please share how this access benefits you. Your story matters. Citation Thomas, Jacob, Catherine A. Lee, and Alan D. Grossman. “A Conserved Helicase Processivity Factor Is Needed for Conjugation and Replication of an Integrative and Conjugative Element.” Ed. Patrick H. Viollier. PLoS Genetics 9.1 (2013). As Published http://dx.doi.org/10.1371/journal.pgen.1003198 Publisher Public Library of Science Version Final published version Citable link http://hdl.handle.net/1721.1/77201 Terms of Use Creative Commons Attribution Detailed Terms http://creativecommons.org/licenses/by/2.5/ A Conserved Helicase Processivity Factor Is Needed for Conjugation and Replication of an Integrative and Conjugative Element Jacob Thomas, Catherine A. Lee, Alan D. Grossman* Department of Biology, Massachusetts Institute of Technology, Cambridge, Massachusetts, United States of America Abstract Integrative and conjugative elements (ICEs) are agents of horizontal gene transfer and have major roles in evolution and acquisition of new traits, including antibiotic resistances. ICEs are found integrated in a host chromosome and can excise and transfer to recipient bacteria via conjugation. Conjugation involves nicking of the ICE origin of transfer (oriT) by the ICE– encoded relaxase and transfer of the nicked single strand of ICE DNA. For ICEBs1 of Bacillus subtilis, nicking of oriT by the ICEBs1 relaxase NicK also initiates rolling circle replication. This autonomous replication of ICEBs1 is critical for stability of the excised element in growing cells. We found a conserved and previously uncharacterized ICE gene that is required for conjugation and replication of ICEBs1. -
Processivity in Early Stages of Transcription by T7 RNA Polymerase?
3966 Biochemistry 1988, 27, 3966-3974 Processivity in Early Stages of Transcription by T7 RNA Polymerase? Craig T. Martin,* Daniel K. Muller,§ and Joseph E. Coleman* Department of Molecular Biophysics and Biochemistry, Yale University, New Haven, Connecticut 0651 0 Received November 24, 1987; Revised Manuscript Received February 10, 1988 ABSTRACT: Immediately following initiation of transcription, T7 RNA polymerase enters a phase in which dissociation of the enzyme-DNA-RNA ternary complex significantly competes with elongation, a process referred to in the Escherichia coli enzyme as abortive cycling [Carpousis, A. J., & Gralla, J. D. (1980) Biochemistry 19, 3245-32531, Characterization of this process in the T7 RNA polymerase system under various reaction conditions and on templates with differing message sequences reveals that conversion to a highly processive ternary complex occurs after incorporation of eight bases and that the relative competition between dissociation and elongation up to this point is influenced by several different forces. In particular, the sequence dependence of abortive falloff suggests that dissociation is favored immediately following incorporation of UMP and is less likely following incorporation of GMP into the RNA message. Abortive cycling is unchanged in transcription from a synthetic oligonucleotide template which is double-stranded in the promoter region but single-stranded throughout the entire message region. This result proves that melting and reannealing of the DNA duplex in the coding region do not contribute to abortive cycling. Furthermore, weakening of promoter binding by an order of magnitude affects abortive cycling only slightly, suggesting that strong interactions with the promoter are not the major cause of abortive cycling. -
Only the Internal B Binding Site of the a Subunit Is Required for Processive Replication by the DNA Polymerase III Holoenzyme
doi:10.1016/j.jmb.2005.04.065 J. Mol. Biol. (2005) 350, 228–239 A Bipartite Polymerase–Processivity Factor Interaction: Only the Internal b Binding Site of the a Subunit is Required for Processive Replication by the DNA Polymerase III Holoenzyme Paul R. Dohrmann and Charles S. McHenry* Department of Biochemistry Previously, we localized the b2 interacting portion of the catalytic subunit and Molecular Genetics (a) of DNA polymerase III to the C-terminal half, downstream of the University of Colorado Health polymerase active site. Since then, two different b2 binding sites within this Sciences Center, 4200 E. Ninth region have been proposed. An internal site includes amino acid residues Ave, B-121, Denver, CO 80262 920–924 (QADMF) and an extreme C-terminal site includes amino acid USA residues 1154–1159 (QVELEF). To permit determination of their relative contributions, we made mutations in both sites and evaluated the biochemical, genetic, and protein binding properties of the mutant a subunits. All purified mutant a subunits retained near wild-type polymerase function, which was measured in non-processive gap-filling assays. Mutations in the internal site abolished the ability of mutant a subunits to participate in processive synthesis. Replacement of the five- residue internal sequence with AAAKK eliminated detectable binding to b2. In addition, mutation of residues required for b2 binding abolished the ability of the resulting polymerase to participate in chromosomal replication in vivo. In contrast, mutations in the C-terminal site exhibited near wild-type phenotypes. a Subunits with the C-terminal site completely removed could participate in processive DNA replication, could bind b2, and, if induced to high level expression, could complement a temperature- sensitive conditional lethal dnaE mutation. -
Mechanism of Type IA Topoisomerases
molecules Review Mechanism of Type IA Topoisomerases Tumpa Dasgupta 1,2,3, Shomita Ferdous 1,2,3 and Yuk-Ching Tse-Dinh 1,2,* 1 Department of Chemistry and Biochemistry, Florida International University, Miami, FL 33199, USA; tdasg002@fiu.edu (T.D.); sferd001@fiu.edu (S.F.) 2 Biomolecular Sciences Institute, Florida International University, Miami, FL 33199, USA 3 Biochemistry PhD Program, Florida International University, Miami, FL 33199, USA * Correspondence: yukching.tsedinh@fiu.edu; Tel.: +1-305-348-4956 Academic Editor: Dagmar Klostermeier Received: 21 September 2020; Accepted: 15 October 2020; Published: 17 October 2020 Abstract: Topoisomerases in the type IA subfamily can catalyze change in topology for both DNA and RNA substrates. A type IA topoisomerase may have been present in a last universal common ancestor (LUCA) with an RNA genome. Type IA topoisomerases have since evolved to catalyze the resolution of topological barriers encountered by genomes that require the passing of nucleic acid strand(s) through a break on a single DNA or RNA strand. Here, based on available structural and biochemical data, we discuss how a type IA topoisomerase may recognize and bind single-stranded DNA or RNA to initiate its required catalytic function. Active site residues assist in the nucleophilic attack of a phosphodiester bond between two nucleotides to form a covalent intermediate with a 50-phosphotyrosine linkage to the cleaved nucleic acid. A divalent ion interaction helps to position the 30-hydroxyl group at the precise location required for the cleaved phosphodiester bond to be rejoined following the passage of another nucleic acid strand through the break.