Subsidence of the Ediacaran Johnnie Formation, Cumulative Offset Along the Lavic Lake Fault, and Geomorphic Surface Development Along The
Total Page:16
File Type:pdf, Size:1020Kb
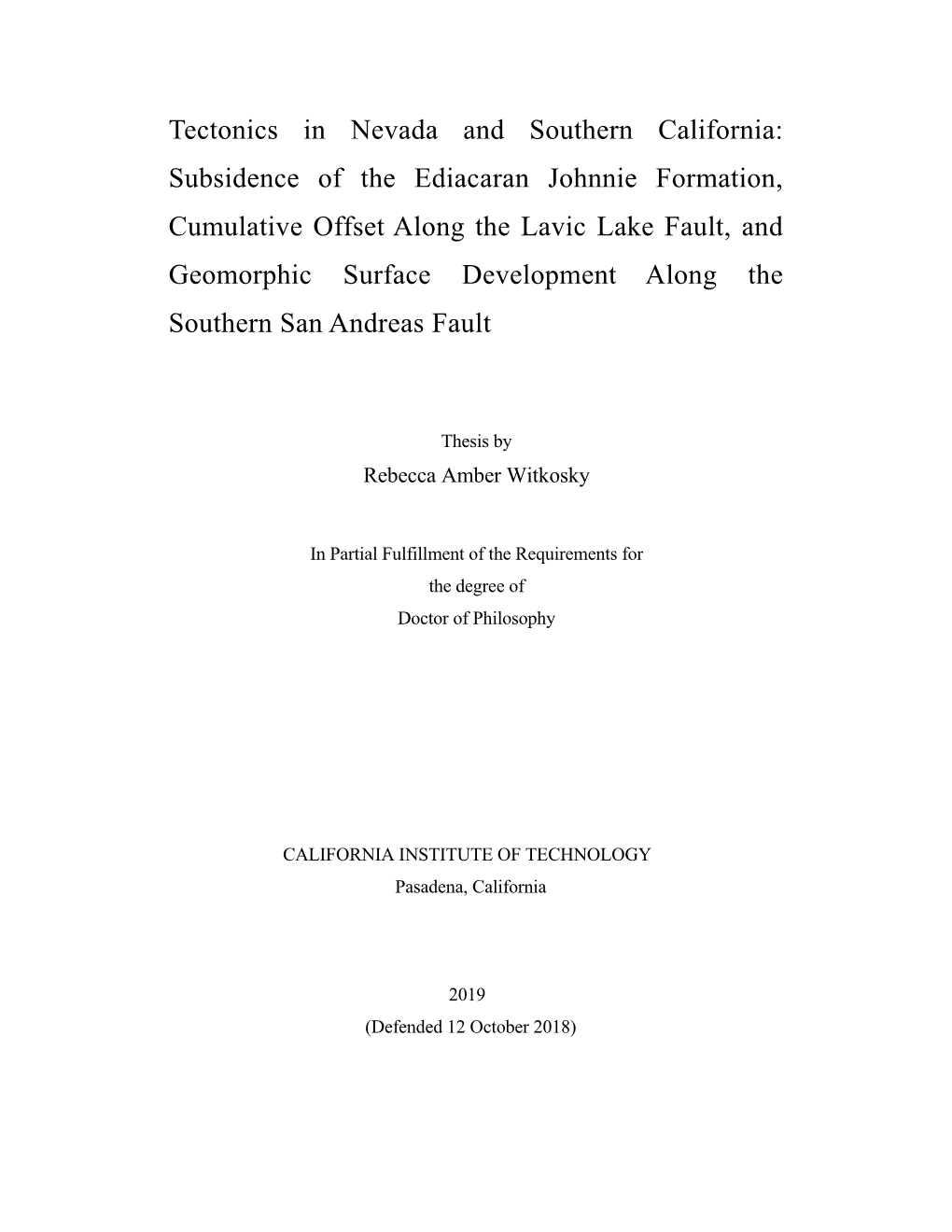
Load more
Recommended publications
-
Mammal Footprints from the Miocene-Pliocene Ogallala
Mammalfootprints from the Miocene-Pliocene Ogallala Formation, easternNew Mexico by ThomasE. Williamsonand SpencerG. Lucas, New Mexico Museum of Natural History and Science,1801 Mountain Road NW Albuquerque, New Mexico 87104-7375 Abstract well-develooed mudcracks. The track- ways are diveloped on the mudstone Mammal trackways preserved in the drape but are preserved as infillings at the Miocene-Pliocene Ogallala Formation of base of the overlying conglomerate (Figs. eastern New Mexico represent the first 2-4). Most tracks are preserved on the report of mammal fossils-from this unit in underside of a single, thick conglomerate New Mexico. These trackwavs are Dre- block (Fig. 3). A few isolated mammal served as infillings in a conglomerate near the base of the Ogallala Formation. At least prints were also observed on the under- four mammalian ichnotaxa are represented, side of adjacent blocks.he depth of the including a single trackway of a large camel infillings suggest that tracks were made in (Gambapessp. A), several prints of an uncer- a relatively soft substrate. Some prints are tain family of artiodactyl (Gambapessp B), a accompanied by marks indicating slip- single trackway of a large feloid carnivoran page on a slick, wet substrate (Fig. 5C). (Bestiopeda sp.), and several indistinct im- Infillings of mudcracks and narrow, cylin- pressions, probably representing more than drical burrows and raindrop impressions one trackway of a small canid carnivoran are Dreserved over some areas of the (Chelipus sp ). The footprints are preserved in a channel-margin facies of an Ogallala tracliway slab. Mammal trackways repre- braided stream. sent at least four ichnotaxa. -
71St Annual Meeting Society of Vertebrate Paleontology Paris Las Vegas Las Vegas, Nevada, USA November 2 – 5, 2011 SESSION CONCURRENT SESSION CONCURRENT
ISSN 1937-2809 online Journal of Supplement to the November 2011 Vertebrate Paleontology Vertebrate Society of Vertebrate Paleontology Society of Vertebrate 71st Annual Meeting Paleontology Society of Vertebrate Las Vegas Paris Nevada, USA Las Vegas, November 2 – 5, 2011 Program and Abstracts Society of Vertebrate Paleontology 71st Annual Meeting Program and Abstracts COMMITTEE MEETING ROOM POSTER SESSION/ CONCURRENT CONCURRENT SESSION EXHIBITS SESSION COMMITTEE MEETING ROOMS AUCTION EVENT REGISTRATION, CONCURRENT MERCHANDISE SESSION LOUNGE, EDUCATION & OUTREACH SPEAKER READY COMMITTEE MEETING POSTER SESSION ROOM ROOM SOCIETY OF VERTEBRATE PALEONTOLOGY ABSTRACTS OF PAPERS SEVENTY-FIRST ANNUAL MEETING PARIS LAS VEGAS HOTEL LAS VEGAS, NV, USA NOVEMBER 2–5, 2011 HOST COMMITTEE Stephen Rowland, Co-Chair; Aubrey Bonde, Co-Chair; Joshua Bonde; David Elliott; Lee Hall; Jerry Harris; Andrew Milner; Eric Roberts EXECUTIVE COMMITTEE Philip Currie, President; Blaire Van Valkenburgh, Past President; Catherine Forster, Vice President; Christopher Bell, Secretary; Ted Vlamis, Treasurer; Julia Clarke, Member at Large; Kristina Curry Rogers, Member at Large; Lars Werdelin, Member at Large SYMPOSIUM CONVENORS Roger B.J. Benson, Richard J. Butler, Nadia B. Fröbisch, Hans C.E. Larsson, Mark A. Loewen, Philip D. Mannion, Jim I. Mead, Eric M. Roberts, Scott D. Sampson, Eric D. Scott, Kathleen Springer PROGRAM COMMITTEE Jonathan Bloch, Co-Chair; Anjali Goswami, Co-Chair; Jason Anderson; Paul Barrett; Brian Beatty; Kerin Claeson; Kristina Curry Rogers; Ted Daeschler; David Evans; David Fox; Nadia B. Fröbisch; Christian Kammerer; Johannes Müller; Emily Rayfield; William Sanders; Bruce Shockey; Mary Silcox; Michelle Stocker; Rebecca Terry November 2011—PROGRAM AND ABSTRACTS 1 Members and Friends of the Society of Vertebrate Paleontology, The Host Committee cordially welcomes you to the 71st Annual Meeting of the Society of Vertebrate Paleontology in Las Vegas. -
Phytoliths of the Barstow Formation Through the Middle Miocene Climatic Optimum: Preliminary Findings Katharine M
Phytoliths of the Barstow Formation through the Middle Miocene Climatic Optimum: preliminary findings Katharine M. Loughney 1,2 and Selena Y. Smith 1, 1 Museum of Paleontology, University of Michigan, 1109 Geddes Ave., Ann Arbor, MI 48109 2 Department of Earth & Environmental Sciences, University of Michigan, 1100 North University Ave., Ann Arbor, MI 48109 abstract—The Middle Miocene Climatic Optimum (MMCO) was an interval of signif- icant warming between 17.0 – 14.0 Ma, and a record of the interval is preserved in its entirety in the type Barstow Formation (19.3 – 13.3 Ma) of southern California. In order to understand the biotic impacts of the MMCO, it is necessary to understand vegetation; however, macrofloral records from the middle Miocene in this region are rare and do not span the MMCO. Phytoliths (plant silica) can be preserved in continental sediments even when macrofossil or pollen remains are not, and they can be diagnostic of specific plant clades and/or functional groups, some of which are useful environmental indica- tors. Sixty-eight sediment samples were collected from 12 stratigraphic sections measured within the Barstow Formation in the Mud Hills, Calico Mountains, and Daggett Ridge, and 39 samples were processed for phytoliths. Ten samples yielded phytoliths, although phytoliths were rare in most of these samples. Paleosols from the uppermost part of the Barstow Formation yielded the most abundant and most diverse phytolith assemblages, including grass bilobates and echinate spheres of palms; grass phytoliths were also identi- fied in samples from the Owl Conglomerate and Middle members but were rare. These phytolith data provide evidence that grasses were present throughout deposition of the Barstow Formation, and that they coexisted with palms in mixed-vegetation habitats. -
Geologic Mapping, Rainbow Basin Field Trip Information
FIELD TRIP 1 Geologic Mapping, Rainbow Basin (Vicinity Barstow, California) Ge 101 Fall Quarter 2013 General Information: We will be departing at 8:00 a.m. on Friday, November 1 from the parking area in front of Arms ("Arms Circle"). Please arrive by 7:45 so there is time to get the trucks packed etc. Attached is a map of how to get to the field area. We will be staying in a motel in Barstow on Friday and Saturday nights (California Inn). We will depart from the motel parking lot for the field area at 8 a.m. on Saturday and Sunday mornings. We will leave the field area at 4 p.m. Sunday, and arrive back at Caltech by 7 p.m. Meals: Rainbow basin is remote and there are no options to purchase food there. We will make a brief stop at a supermarket in Barstow Friday morning to purchase lunch food, snacks and drinks. You may however opt to bring your own food and pack it in ice chests before we leave, up to and including three lunches, two breakfasts and two dinners. Prof. and TAs usually opt for breakfast at Carrows (a short drive from the motel) at 7 a.m., but the California Inn has a free breakfast. There are lots of restaurant options for dinner, from McDonalds up to various moderately priced restaurants. If you opt to purchase lunch food Friday morning in Barstow and otherwise eat in restaurants, you should budget about $80-100. Report: The field report will be an inked and colored geologic map with a map legend and cross section, plus a short description of the geologic history of the map area, listing events depicted on the geologic map, plus additional information compiled in Lab. -
Structural and Stratigraphic Evolution of the Calico Mountains
Structural and stratigraphic evolution of the Calico Mountains: Implications for early Miocene extension and Neogene transpression in the central Mojave Desert, California John S. Singleton* Phillip B. Gans Department of Earth Science, University of California, Santa Barbara, California 93106, USA ABSTRACT rapid unroofi ng of the central Mojave meta- (the Waterman Hills detachment fault) that morphic core complex, yet extension in the juxtaposes tilted early Miocene volcanic and New geologic mapping, structural data, Calico Mountains is minor and is overprinted sedimentary rocks in the hanging wall against and 40Ar/39Ar geochronology document early by dextral faulting and transpression. variably mylonitized basement rocks in the Miocene sedimentation and volcanism and Calico Member beds north of the Calico footwall. Based on apparent offsets of pre-Ter- Neogene deformation in the Calico Moun- fault are intensely folded into numerous tiary markers, several workers (Glazner et al., tains, located in a complexly deformed region east-west–trending, upright anticlines and 1989; Walker et al., 1990; Martin et al., 1993) of California’s central Mojave Desert. Across synclines that represent 25%–33% (up to proposed that 40–60 km of northeast-directed most of the Calico Mountains, volcaniclastic ~0.5 km) north-south shortening. Folds are normal slip occurred along the Waterman Hills sediments and dacitic rocks of the Pickhan- detached along the base of the Calico Mem- detachment fault. The distribution of exten- dle Formation accumulated rapidly between ber and thrust over the Pickhandle Forma- sion is controversial. Dokka (1989) argued that ca. 19.4 and 19 Ma. Overlying fi ne-grained tion, which dips homoclinally ~15–30°S to regional extension occurred within an east- lacustrine beds (here referred to as the Cal- SE. -
Tr-98-04 30-26
SE9900227 Technical Report TR-98-04 Volume I MAQARIN natural analogue study: Phase III Edited by J A T Smellie December 1998 Svensk Karnbranslehantering AB Swedish Nuclear Fuel and Waste Management Co Box 5864 SE-102 40 Stockholm Sweden Tel 08-459 84 00 +46 8 459 84 00 Fax 08-661 57 19 +46 8 661 57 19 30-26 MAQARIN natural analogue study: Phase III Edited by J.A.T. Smellie December 1998 VOLUME!: Chapters 1-13 VOLUME II: Appendices A-R This report concerns a study which was conducted for SKB. The conclusions and viewpoints presented in the report are those of the author(s) and do not necessarily coincide with those of the client. Information on SKB technical reports from 1977-1978 (TR 121), 1979 (TR 79-28), 1980 (TR 80-26), 1981 (TR 81-17), 1982 (TR 82-28), 1983 (TR 83-77), 1984 (TR 85-01), 1985 (TR 85-20), 1986 (TR 86-31), 1987 (TR 87-33), 1988 (TR 88-32), 1989 (TR 89-40), 1990 (TR 90-46), 1991 (TR 91-64), 1992 (TR 92-46), 1993 (TR 93-34), 1994 (TR 94-33), 1995 (TR 95-37) and 1996 (TR 96-25) is available through SKB. m^ffm?^ AKE TIBERIAS Qarfah , . /olbta' ? / .-V- ) had Set L^ Shafeni ," • '., Haiyan' /""•' ,. ^_ .I-...-- oel. MuiSti £1 Husetntya /..'" oHaiyan '• o £1 Jazza umman ,.-;p"i>EI Majarra A^ Ucn Pd Oananir t-^jAb'u Nuseir ,•"' ' ftaiib ..;•-•—.. | Jcbel el Adorn ; ,.-",' -•\' °Hawwara"-. / \ Bartholomew 1995 \ Reproduced with kind permission \ . , .,„ , . ''..\A::;..._ \ ««* '''a, •' / ACKNOWLEDGEMENTS his study has been funded by Nagra (Switzerland), Nirex (U.K.), SKB (Sweden) Tand the Environment Agency (U.K.); their active support is gratefully acknowledged. -
Mojave Miocene Robert E
Mojave Miocene Robert E. Reynolds, editor California State University Desert Studies Center 2015 Desert Symposium April 2015 Front cover: Rainbow Basin syncline, with rendering of saber cat by Katura Reynolds. Back cover: Cajon Pass Title page: Jedediah Smith’s party crossing the burning Mojave Desert during the 1826 trek to California by Frederic Remington Past volumes in the Desert Symposium series may be accessed at <http://nsm.fullerton.edu/dsc/desert-studies-center-additional-information> 2 2015 desert symposium Table of contents Mojave Miocene: the field trip 7 Robert E. Reynolds and David M. Miller Miocene mammal diversity of the Mojave region in the context of Great Basin mammal history 34 Catherine Badgley, Tara M. Smiley, Katherine Loughney Regional and local correlations of feldspar geochemistry of the Peach Spring Tuff, Alvord Mountain, California 44 David C. Buesch Phytoliths of the Barstow Formation through the Middle Miocene Climatic Optimum: preliminary findings 51 Katharine M. Loughney and Selena Y. Smith A fresh look at the Pickhandle Formation: Pyroclastic flows and fossiliferous lacustrine sediments 59 Jennifer Garrison and Robert E. Reynolds Biochronology of Brachycrus (Artiodactyla, Oreodontidae) and downward relocation of the Hemingfordian– Barstovian North American Land Mammal Age boundary in the respective type areas 63 E. Bruce Lander Mediochoerus (Mammalia, Artiodactyla, Oreodontidae, Ticholeptinae) from the Barstow and Hector Formations of the central Mojave Desert Province, southern California, and the Runningwater and Olcott Formations of the northern Nebraska Panhandle—Implications of changes in average adult body size through time and faunal provincialism 83 E. Bruce Lander Review of peccaries from the Barstow Formation of California 108 Donald L. -
Paleontological Resources
Draft DRECP and EIR/EIS CHAPTER III.10. PALEONTOLOGICAL RESOURCES III.10 PALEONTOLOGICAL RESOURCES A paleontological resource is defined in the federal Paleontological Resources Preservation Act (PRPA) as the “fossilized remains, traces, or imprints of organisms, preserved in or on the earth’s crust, that are of paleontological interest and that provide information about the history of life on earth” (16 United States Code [U.S.C.] 470aaa[1][c]). For the purpose of this analysis, a significant paleontological resource is “considered to be of scientific interest, including most vertebrate fossil remains and traces, and certain rare or unusual inverte- brate and plant fossils. A significant paleontological resource is considered to be scientifically important for one or more of the following reasons: It is a rare or previously unknown species It is of high quality and well preserved It preserves a previously unknown anatomical or other characteristic It provides new information about the history of life on earth It has identified educational or recreational value. Paleontological resources that may be considered not to have paleontological significance include those that lack provenance or context, lack physical integrity because of decay or natural erosion, or are overly redundant or otherwise not useful for academic research” (Bureau of Land Management [BLM] Instruction Memorandum [IM] 2009-011; included in Appendix R2). The intrinsic value of paleontological resources largely stems from the fact that fossils serve as the only direct evidence of prehistoric life. They are thus used to understand the history of life on Earth, the nature of past environments and climates, the biological mem- bership and structure of ancient ecosystems, and the pattern and process of organic evolution and extinction. -
Palaeontologia Electronica Microtoid Cricetids and the Early History Of
Palaeontologia Electronica http://palaeo-electronica.org Microtoid cricetids and the early history of arvicolids (Mammalia, Rodentia) Oldrich Fejfar, Wolf-Dieter Heinrich, Laszlo Kordos, and Lutz Christian Maul ABSTRACT In response to environmental changes in the Northern hemisphere, several lines of brachyodont-bunodont cricetid rodents evolved during the Late Miocene as “micro- toid cricetids.” Major evolutionary trends include increase in the height of cheek tooth crowns and development of prismatic molars. Derived from a possible Megacricetodon or Democricetodon ancestry, highly specialised microtoid cricetids first appeared with Microtocricetus in the Early Vallesian (MN 9) of Eurasia. Because of the morphological diversity and degree of parallelism, phylogenetic relationships are difficult to detect. The Trilophomyinae, a more aberrant cricetid side branch, apparently became extinct without descendants. Two branches of microtoid cricetids can be recognized that evolved into “true” arvicolids: (1) Pannonicola (= Ischymomys) from the Late Vallesian (MN 10) to Middle Turolian (MN 12) of Eurasia most probably gave rise to the ondatrine lineage (Dolomys and Propliomys) and possibly to Dicrostonyx, whereas (2) Microt- odon known from the Late Turolian (MN 13) and Early Ruscinian (MN 14) of Eurasia and possibly parts of North America evolved through Promimomys and Mimomys eventually to Microtus, Arvicola and other genera. The Ruscinian genus Tobienia is presumably the root of Lemmini. Under this hypothesis, in contrast to earlier views, two evolutionary sources of arvicolids would be taken into consideration. The ancestors of Pannonicola and Microtodon remain unknown, but the forerunner of Microtodon must have had a brachyodont-lophodont tooth crown pattern similar to that of Rotundomys bressanus from the Late Vallesian (MN 10) of Western Europe. -
Index to the Geologic Names of North America
Index to the Geologic Names of North America GEOLOGICAL SURVEY BULLETIN 1056-B Index to the Geologic Names of North America By DRUID WILSON, GRACE C. KEROHER, and BLANCHE E. HANSEN GEOLOGIC NAMES OF NORTH AMERICA GEOLOGICAL SURVEY BULLETIN 10S6-B Geologic names arranged by age and by area containing type locality. Includes names in Greenland, the West Indies, the Pacific Island possessions of the United States, and the Trust Territory of the Pacific Islands UNITED STATES GOVERNMENT PRINTING OFFICE, WASHINGTON : 1959 UNITED STATES DEPARTMENT OF THE INTERIOR FRED A. SEATON, Secretary GEOLOGICAL SURVEY Thomas B. Nolan, Director For sale by the Superintendent of Documents, U.S. Government Printing Office Washington 25, D.G. - Price 60 cents (paper cover) CONTENTS Page Major stratigraphic and time divisions in use by the U.S. Geological Survey._ iv Introduction______________________________________ 407 Acknowledgments. _--__ _______ _________________________________ 410 Bibliography________________________________________________ 410 Symbols___________________________________ 413 Geologic time and time-stratigraphic (time-rock) units________________ 415 Time terms of nongeographic origin_______________________-______ 415 Cenozoic_________________________________________________ 415 Pleistocene (glacial)______________________________________ 415 Cenozoic (marine)_______________________________________ 418 Eastern North America_______________________________ 418 Western North America__-__-_____----------__-----____ 419 Cenozoic (continental)___________________________________ -
In Search of Vanished Ages--Field Trips to Fossil Localities in California, Nevada, and Utah
i In Search Of Vanished Ages Field trips to fossil localities in California, Nevada, and Utah By Inyo A view across the middle Miocene Barstow Formation on California’s Mojave Desert. Here, limestone concretions that occur in rocks deposited in a freshwater lake system approximately 17 million year-ago produce exquisitely preserved, fully three-dimensional insects, spiders, water mites, and fairy shrimp that can be dissolved free of their stone encasings with a diluted acid solution—one of only a handful of localities worldwide where fossil insects can be removed successfully from their matrixes without obliterating the specimens. ii Table of Contents Chapter Page 1—Fossil Plants At Aldrich Hill 1 2—A Visit To Ammonite Canyon, Nevada 6 3—Fossil Insects And Vertebrates On The Mojave Desert, California 15 4—Fossil Plants At Buffalo Canyon, Nevada 45 5--Ordovician Fossils At The Great Beatty Mudmound, Nevada 50 6--Fossil Plants And Insects At Bull Run, Nevada 58 7-- Field Trip To The Copper Basin Fossil Flora, Nevada 65 8--Trilobites In The Nopah Range, Inyo County, California 70 9--Field Trip To A Vertebrate Fossil Locality In The Coso Range, California 76 10--Plant Fossils In The Dead Camel Range, Nevada 83 11-- A Visit To The Early Cambrian Waucoba Spring Geologic Section, California 88 12-- Fossils In Millard County, Utah 95 13--A Visit To Fossil Valley, Great Basin Desert, Nevada 107 14--High Inyo Mountains Fossils, California 119 15--Early Cambrian Fossils In Western Nevada 126 16--Field Trip To The Kettleman Hills Fossil District, -
The Miocene: the Future of the Past
Research Collection Journal Article The Miocene: The Future of the Past Author(s): Steinthorsdottir, Margret; Stoll, Heather; et al. Publication Date: 2021-04 Permanent Link: https://doi.org/10.3929/ethz-b-000483186 Originally published in: Paleoceanography and Paleoclimatology 36(4), http://doi.org/10.1029/2020PA004037 Rights / License: Creative Commons Attribution-NonCommercial-NoDerivatives 4.0 International This page was generated automatically upon download from the ETH Zurich Research Collection. For more information please consult the Terms of use. ETH Library RESEARCH ARTICLE The Miocene: The Future of the Past 10.1029/2020PA004037 M. Steinthorsdottir1,2 , H. K. Coxall2,3, A. M. de Boer2,3 , M. Huber4 , N. Barbolini2,5 , C. D. Bradshaw6,7 , N. J. Burls8 , S. J. Feakins9 , E. Gasson10 , J. Henderiks11 , Special Section: A. E. Holbourn12, S. Kiel1,2 , M. J. Kohn13 , G. Knorr14, W. M. Kürschner15, C. H. Lear16 , The Miocene: The Future of D. Liebrand17, D. J. Lunt10 , T. Mörs1,2 , P. N. Pearson16 , M. J. Pound18 , H. Stoll19, and the Past C. A. E. Strömberg20 Key Points: 1Department of Palaeobiology, Swedish Museum of Natural History, Stockholm, Sweden, 2Bolin Centre for Climate • Miocene floras, faunas, and Research, Stockholm University, Stockholm, Sweden, 3Department of Geological Sciences, Stockholm University, paleogeography were similar to 4 today and provide plausible analogs Stockholm, Sweden, Department of Earth, Atmospheric and Planetary Sciences, Purdue University, West Lafayette, 5 for future climatic warming IN, USA,