Effect of the Functional Diversity of Communities of Herbivorous Insects on the Diversity and Functioning of a Grassland Ecosystem Hélène Deraison
Total Page:16
File Type:pdf, Size:1020Kb
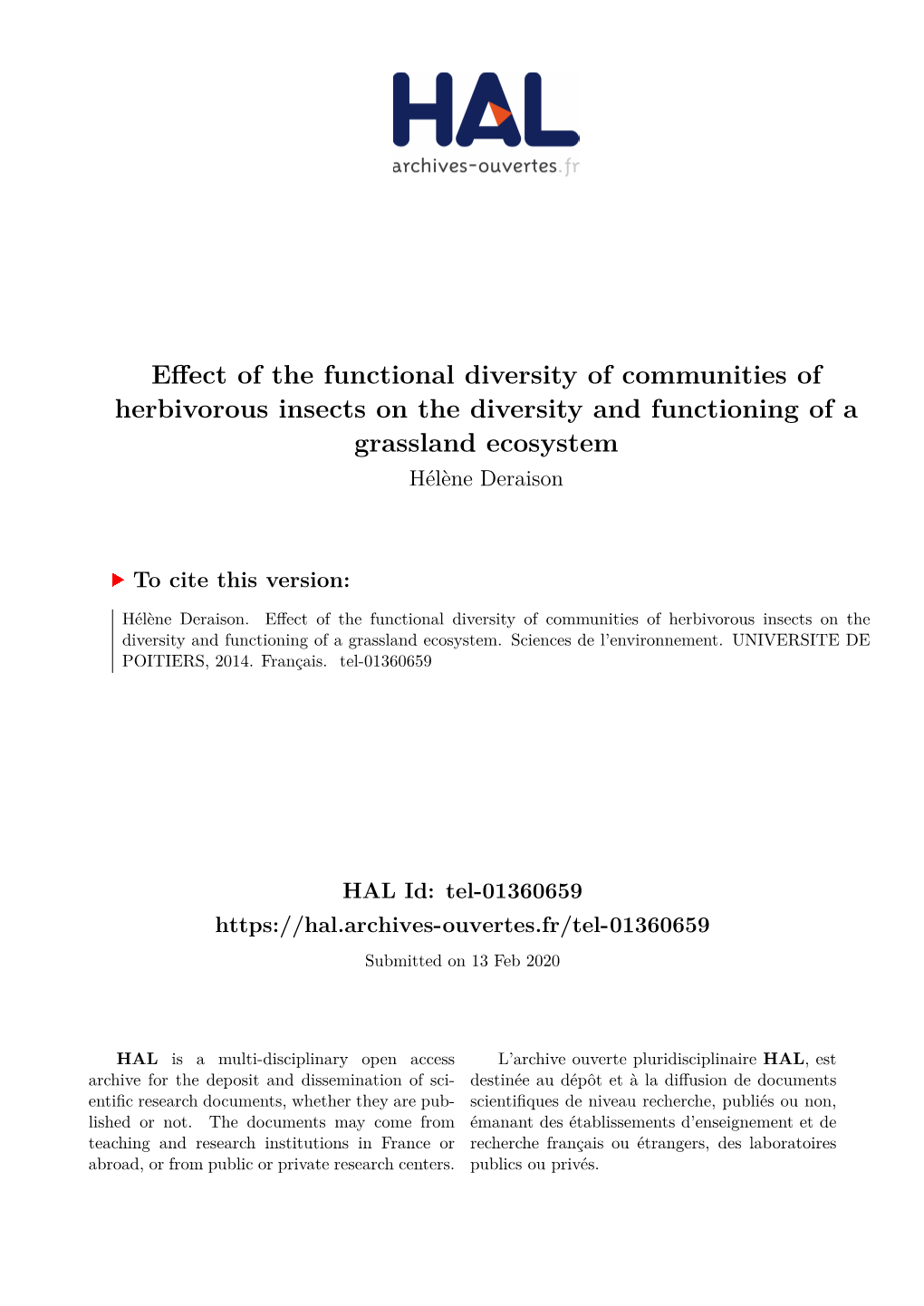
Load more
Recommended publications
-
Nouvelles Données Taxonomiques Et Chorologiques Sur Aiolopus Puissanti Defaut Et A
Matériaux Orthoptériques et Entomocénotiques, 13, 2008 : 5-23 Nouvelles données taxonomiques et chorologiques sur Aiolopus puissanti Defaut et A. thalassinus (F.) (Orthoptera, Acrididae). 1 2 Bernard DEFAUT & Stéphane JAULIN 1 ASCETE, Aynat, 09400 Bédeilhac-et-Aynat <[email protected]> 2 OPIE-LR, Digue d’Orry, avenue Torcatis, 66000 Perpignan <[email protected]> Résumé. Les auteurs ont mesuré ou calculé 33 paramètres biométriques chez 133 mâles, et 28 paramètres chez 136 femelles d’Aiolopus groupe thalassinus provenant du domaine paléarctique occidental. Des analyses en composantes principales et des dia- grammes de dispersion ont été établis, qui permettent de préciser les caractères distinctif d’A. puissanti Defaut par rapport à A. tha- lassinus (F.), et de proposer une nouvelle sous-espèce d’A. thalassinus en Corse. Des cartes de répartition géographique des deux es- pèces sont présentées. Mots clés. Acrididae, Aiolopus puissanti Defaut ; Aiolopus thalassinus (F.) ; domaine paléarctique occidental ; Abstract. The authors measured or calculated 33 biometric parameters in 133 males, and 28 parameters in 136 females of Aiolopus group thalassinus coming from the Western palearctic region. Principal component analyses and scatter diagrams were established, which make it possible to specify the characters distinctive of A. puissanti Defaut compared to A. thalassinus (F.), and to propose a new subspecies of A. thalassinus for Corsica. Geographical distribution maps of the two species are presented. Key Words. Acrididae, Aiolopus puissanti Defaut ; Aiolopus thalassinus (F.) ; Western palearctic region. –oOo– INTRODUCTION Longueur du sillon sous-oculaire (Sso). Largeur minimale du vertex, entre le bord externe des ca- Les quatre espèces d’Aiolopus présentes dans le rènes latérales (ou entre leur prolongement immédiat) domaine paléarctique occidental se répartissent en deux (v). -
Biochemical Features of Some Insects Species Potential Preys of Predators 3
International Journal of Bio-Technology and Research (IJBTR) ISSN(P): 2249-6858; ISSN(E): 2249-796X Vol. 4, Issue 4, Aug 2014, 1-8 © TJPRC Pvt. Ltd. BIOCHEMICAL FEATURES OF SOME INSECTS SPECIES POTENTIAL PREYS OF PREDATORS NADIA YAHIA1 & BELKACEM BAZIZ, SALAHEDDINE DOUMANDJI2 1Agronomic National Upper School El-Harrach, Algiers, Algeria 2Ornithology Laboratory, Agronomic National Upper School EL-Harrach, Algeria ABSTRACT Mower- net is the technical sampling used on the ground relating to study arthropoda in experimental plots, and in gardens of Agronomic National School El Harrach. Insects category dominates with 1.120 individuals (97.1%) and 55% species (94.7%).Orthoptera are highly mentioned with a number of 856 individuals (74.2%). Gastropoda, Crustacea and Myriapoda, together correspond hardly to 2.9% according to total of captured individuals. Three techniques of biochemical analyses are used in laboratory with the aim to determine nutritive values and so energetic contribution of some insects species considered as potential preys of different predators. Used methods in the present work are the Kjeldhal method for dose ot total nitrogen, Soxhlet method for total lipids and Bertrand method relating to sugars. This analysis shows that proteins rates found in different species vary between 3.5 and 28.4%. For sugars rates found, the weaker is 3.4% while the stronger is 25.5%. For lipids, the recorded contents are about 9.8 and 33.3%. The total energetic contribution brought by the three biochemical components which are, proteins,lipids,and total sugars is estimated at 4.56 Kcal by female of Aiolopus thalassinus until 13.77 Kcal by male of Aiolopus strepens. -
Catalogue of the Type Specimens Deposited in the Department of Entomology, National Museum, Prague, Czech Republic*
ACTA ENTOMOLOGICA MUSEI NATIONALIS PRAGAE Published 30.iv.2014 Volume 54(1), pp. 399–450 ISSN 0374-1036 http://zoobank.org/urn:lsid:zoobank.org:pub:7479D174-4F1D-4465-9EEA-2BBB5E1FC2A2 Catalogue of the type specimens deposited in the Department of Entomology, National Museum, Prague, Czech Republic* Polyneoptera Lenka MACHÁýKOVÁ & Martin FIKÁýEK Department of Entomology, National Museum in Prague, Kunratice 1, CZ-148 00 Praha 4-Kunratice, Czech Republic & Department of Zoology, Faculty of Sciences, Charles University in Prague, Viniþná 7, CZ-128 43, Praha 2, Czech Republic; e-mails: [email protected]; m¿ [email protected] Abstract. Type specimens from the collection of the polyneopteran insect orders (Dermaptera, Blattodea, Orthoptera, Phasmatodea) deposited in the Department of Entomology, National Museum, Prague are catalogued. We provide precise infor- mation about types of 100 taxa (5 species of Dermaptera, 3 species of Blattodea, 4 species of Phasmatodea, 55 species of Caelifera, and 33 species of Ensifera), including holotypes of 38 taxa. The year of publication of Calliptamus tenuicer- cis anatolicus MaĜan, 1952 and Calliptamus tenuicercis iracus MaĜan, 1952 are corrected. The authorship of the names traditionally ascribed to J. Obenberger is discussed in detail. Only the name Podisma alpinum carinthiacum Obenberger, 1926 is available since the publication by OBENBERGER (1926a). ‘Stenobothrus (Stauroderus) biguttulus ssp. bicolor Charp. 1825’ and ‘Stenobothrus (Stau- roderus) ssp. collinus Karny’ sensu OBENBERGER (1926a,b) refer to Gryllus bicolor Charpentier, 1825 and Stauroderus biguttulus var. collina Karny, 1907, respectively, which both have to be considered available already since their original descriptions by CHARPENTIER (1825) and KARNY (1907). Key words. -
Blauflüglige Ödlandschrecke (Oedipoda Caerulescens) (Stand November 2011)
Niedersächsische Strategie zum Arten- und Biotopschutz Vollzugshinweise zum Schutz von Wirbellosenarten in Niedersachsen Wirbellosenarten mit Priorität für Erhaltungs- und Entwicklungsmaßnahmen Blauflüglige Ödlandschrecke (Oedipoda caerulescens) (Stand November 2011) Inhalt 1 Lebensweise und Lebensraum 3 Erhaltungsziele 1.1 Merkmale, Lebensweise 4 Maßnahmen 1.2 Lebensraumansprüche 4.1 Schutz- und Entwicklungsmaßnahmen 2 Bestandssituation und Verbreitung 4.2 Gebiete für die Umsetzung mit 2.1 Verbreitung in Niedersachsen Prioritätensetzung 2.2 Bestandssituation in Deutschland und 4.3 Bestandsüberwachung Niedersachsen 5 Schutzinstrumente 2.3 Schutzstatus 6 Literatur 2.4 Erhaltungszustand 2.5 Beeinträchtigungen und Gefährdungen Abb. 1: Blauflüglige Ödlandschrecke (Foto: W. Höxter) Niedersächsischer Landesbetrieb für Wasserwirtschaft, Küsten- und Naturschutz – NLWKN 1 Niedersächsische Strategie zum Arten- und Biotopschutz – Vollzugshinweise Wirbellosenarten – Blauflüglige Ödlandschrecke Oedipoda caerulescens (prioritär) November 2011 1 Lebensweise und Lebensraum 1.1 Merkmale, Lebensweise . Die Blauflüglige Ödlandschrecke (Oedipoda caerulescens) ist eine Art aus der Familie der Acrididae (Feldheuschrecken). Sehr variable, dem Untergrund angepasste Grundfarbe . Wird gelegentlich mit Sphingonotus caerulans verwechselt: Unterscheidung: blaue Hinter- flügel mit deutlicher Hinterflügelbinde, gewölbtes Halsschild und eine Stufe am Oberrand des Hinterschenkels . Gelegentlich Individuen mit rötlich gefärbten Flügeln, die mit der Rotflügligen Ödlandschre- -
ARTICULATA 2010 25 (1): 73–107 FAUNISTIK Orthoptera and Mantodea in the Collection of the Macedonian Museum of Natural Histo
Deutschen Gesellschaft für Orthopterologie e.V.; download http://www.dgfo-articulata.de/ ARTICULATA 2010 25 (1): 73107 FAUNISTIK Orthoptera and Mantodea in the collection of the Macedonian Museum of Natural History (Skopje) with an annotated check-list of the groups in Macedonia Dragan P. Chobanov & Branislava Mihajlova Abstract During the revision of the Orthoptera collection of the Macedonian Museum of Natural History (Skopje) in 2004, four Mantodea and 102 Orthoptera species (al- together 1057 specimens) collected in the Republic of Macedonia were deter- mined. Furthermore, a revision of the literature about the Macedonian orthopte- ran fauna was executed and some own unpublished records were added. As a result of the present study, eight taxa are added and 27 taxa are subtracted from the list of the Macedonian fauna. Thus, the updated check list of the Orthoptera fauna of Macedonia comprises four species of Mantodea, 167 Orthoptera taxa and two additional subspecies. The study also revealed that two Orthoptera spe- cies must be eliminated from the list of the Serbian fauna. Zusammenfassung Im Zuge der Bearbeitung der Orthopterensammlung des Naturhistorischen Mu- seums von Mazedonien (Skopje), im Jahre 2004, konnten vier Mantiden- und 102 Orthopterenarten (zusammen 1057 Individuen), die in der Republik Mazedo- nien gesammelt wurden, untersucht werden. Zusätzlich wurde eine umfangreiche Literaturstudie über die mazedonische Orthopterenfauna durchgeführt, die weite- re Nachweise lieferte. Die vorliegende Studie ergab acht neue Taxa für Mazedo- nien, wohingegen 27 der für die mazedonische Fauna angegebenen Taxa nicht in Mazedonien vorkommen. Die aktuelle Checkliste der Orthopterenfauna Maze- doniens enthält somit vier Arten Gottesanbeterinnen, 167 Heuschreckenarten und zwei weitere Unterarten. -
Observations Récentes D'aiolopus Strepens (Latreille, 1804) Dans Le
Matériaux orthoptériques et entomocénotiques, 2018, 23 : 101-105 Observations récentes d’Aiolopus strepens (Latreille, 1804) dans le Massif armoricain deux-sévrien (Orthoptera, Caelifera, Acrididae, Locustinae) 1 2 Guy-Noël YOU & Florian DORÉ 1 : 20 Allée de la Vannelière - 79140 Cerizay [[email protected]] 2 : Deux-Sèvres Nature Environnement, 48 Rue Rouget de Lisle – 79000 Niort [[email protected]] Résumé. Aiolopus strepens est un criquet thermophile à répartition méridionale qui affectionne une large gamme de milieux dans le Sud de la France. Jusqu’en 2010, il est considéré rare en Deux-Sèvres et non connu du Massif armoricain. Depuis, la mise en place d’un atlas régional en Poitou-Charentes a suscité un certain engouement. Aiolopus strepens est désormais connu du Sud des Deux-Sèvres, où il est assez commun, au Nord, où il est plus rare. Suite à nos constats, nous pouvons probablement avancer que l'espèce s'est installée sur des sites où elle n'était pas connue avant 2016. Ceci met en avant une expansion actuelle de l’espèce vers le nord de la France comme en témoigne les observations dans d’autres départements. Mots clés. Aiolopus strepens ; expansion ; Deux-Sèvres. Abstract. Aiolopus strepens is a thermophilic grasshopper with southern distribution occupying a wide range of habitats in the South of France. Until 2010, this species is considered rare in Deux-Sèvres and unknown in the Armorican massif. Since then, the establishment of a regional atlas of Orthoptera in Poitou-Charentes has generated a certain enthusiasm. Aiolopus strepens is now known quite common in Southern Deux-Sèvres and rarer to the North. -
Landscape-Scale Connections Between the Land Use, Habitat Quality and Ecosystem Goods and Services in the Mureş/Maros Valley
TISCIA monograph series Landscape-scale connections between the land use, habitat quality and ecosystem goods and services in the Mureş/Maros valley Edited by László Körmöczi Szeged-Arad 2012 Two countries, one goal, joint success! Landscape-scale connections between the land use, habitat quality and ecosystem goods and services in the Mureş/Maros valley TISCIA monograph series 1. J. Hamar and A. Sárkány-Kiss (eds.): The Maros/Mureş River Valley. A Study of the Geography, Hydrobiology and Ecology of the River and its Environment, 1995. 2. A. Sárkány-Kiss and J. Hamar (eds.): The Criş/Körös Rivers’ Valleys. A Study of the Geography, Hydrobiology and Ecology of the River and its Environment, 1997. 3. A. Sárkány-Kiss and J. Hamar (eds.): The Someş/Szamos River Valleys. A Study of the Geography, Hydrobiology and Ecology of the River and its Environment, 1999. 4. J. Hamar and A. Sárkány-Kiss (eds.): The Upper Tisa Valley. Preparatory Proposal for Ramsar Site Designation and an Ecological Background, 1999. 5. L. Gallé and L. Körmöczi (eds.): Ecology of River Valleys, 2000. 6. Sárkány-Kiss and J. Hamar (eds.): Ecological Aspects of the Tisa River Basin, 2002. 7. L. Gallé (ed.): Vegetation and Fauna of Tisza River Basin, I. 2005. 8. L. Gallé (ed.): Vegetation and Fauna of Tisza River Basin, II. 2008. 9. L. Körmöczi (ed.): Ecological and socio-economic relations in the valleys of river Körös/Criş and river Maros/Mureş, 2011. 10. L. Körmöczi (ed.): Landscape-scale connections between the land use, habitat quality and ecosystem goods and services in the Mureş/Maros valley, 2012. -
Grasshoppers and Locusts (Orthoptera: Caelifera) from the Palestinian Territories at the Palestine Museum of Natural History
Zoology and Ecology ISSN: 2165-8005 (Print) 2165-8013 (Online) Journal homepage: http://www.tandfonline.com/loi/tzec20 Grasshoppers and locusts (Orthoptera: Caelifera) from the Palestinian territories at the Palestine Museum of Natural History Mohammad Abusarhan, Zuhair S. Amr, Manal Ghattas, Elias N. Handal & Mazin B. Qumsiyeh To cite this article: Mohammad Abusarhan, Zuhair S. Amr, Manal Ghattas, Elias N. Handal & Mazin B. Qumsiyeh (2017): Grasshoppers and locusts (Orthoptera: Caelifera) from the Palestinian territories at the Palestine Museum of Natural History, Zoology and Ecology, DOI: 10.1080/21658005.2017.1313807 To link to this article: http://dx.doi.org/10.1080/21658005.2017.1313807 Published online: 26 Apr 2017. Submit your article to this journal View related articles View Crossmark data Full Terms & Conditions of access and use can be found at http://www.tandfonline.com/action/journalInformation?journalCode=tzec20 Download by: [Bethlehem University] Date: 26 April 2017, At: 04:32 ZOOLOGY AND ECOLOGY, 2017 https://doi.org/10.1080/21658005.2017.1313807 Grasshoppers and locusts (Orthoptera: Caelifera) from the Palestinian territories at the Palestine Museum of Natural History Mohammad Abusarhana, Zuhair S. Amrb, Manal Ghattasa, Elias N. Handala and Mazin B. Qumsiyeha aPalestine Museum of Natural History, Bethlehem University, Bethlehem, Palestine; bDepartment of Biology, Jordan University of Science and Technology, Irbid, Jordan ABSTRACT ARTICLE HISTORY We report on the collection of grasshoppers and locusts from the Occupied Palestinian Received 25 November 2016 Territories (OPT) studied at the nascent Palestine Museum of Natural History. Three hundred Accepted 28 March 2017 and forty specimens were collected during the 2013–2016 period. -
Extra Nucleolar Activity Associated with Chromosome Segment in The
Heredity 56 (1986) 237—241 The Genetical Society of Great Britain Received 22 July 1985 Extra nucleolar activity associated with presence of a supernumerary chromosome segment in the grasshopper Oedipoda fuscocincta J. P. M. Camacho, J. Navas-Castillo Departamento de Genética, Facultad de Ciencias, and J. Cabrero Universidad de Granada, 18071 Granada, Spain. The standard males of Oedipoda fuscocincta show a single active nucleolus organiser region (NOR) located in the secondary constriction of the M9 chromosomes. Nine males from three different populations proved to be heterozygous for a heterochromatic supernumerary segment which is distally located on the S10 chromosome. This also showed NOR activity. All available data seem to support the hypothesis that this extra segment on the S10 chromosome has arisen by translocation of part of the M9 chromosome, specifically that located between the centromere and the secondary constriction. INTRODUCTION However, in this paper we report a supernu- merary chromosome segment containing an active Oneform of chromosomal polymorphism com- NOR, which may serve as evidence in favour of monly identified in acridid grasshoppers is that the origin of this extra segment by means of translo- caused by the presence of extra chromatin seg- cation. ments attached one or more chromosomes of the standard complement. That is to say they are super- numerary chromosome segments. The majority of MATERIALSAND METHODS observed extra segments are heterochromatic in nature, although two cases of euchromatic ones Forty-sevenadult males of the grasshopper have been recorded in the gomphocerine grasshop- Oedipoda fuscocincta Saussure were caught at five pers namely, Omocestus bolivari (Camacho et a!., localities in the South of the Iberian Peninsula, 1984) and Chorthippus binotatus (Cabrero, 1985). -
Arthropods in Linear Elements
Arthropods in linear elements Occurrence, behaviour and conservation management Thesis committee Thesis supervisor: Prof. dr. Karlè V. Sýkora Professor of Ecological Construction and Management of Infrastructure Nature Conservation and Plant Ecology Group Wageningen University Thesis co‐supervisor: Dr. ir. André P. Schaffers Scientific researcher Nature Conservation and Plant Ecology Group Wageningen University Other members: Prof. dr. Dries Bonte Ghent University, Belgium Prof. dr. Hans Van Dyck Université catholique de Louvain, Belgium Prof. dr. Paul F.M. Opdam Wageningen University Prof. dr. Menno Schilthuizen University of Groningen This research was conducted under the auspices of SENSE (School for the Socio‐Economic and Natural Sciences of the Environment) Arthropods in linear elements Occurrence, behaviour and conservation management Jinze Noordijk Thesis submitted in partial fulfilment of the requirements for the degree of doctor at Wageningen University by the authority of the Rector Magnificus Prof. dr. M.J. Kropff, in the presence of the Thesis Committee appointed by the Doctorate Board to be defended in public on Tuesday 3 November 2009 at 1.30 PM in the Aula Noordijk J (2009) Arthropods in linear elements – occurrence, behaviour and conservation management Thesis, Wageningen University, Wageningen NL with references, with summaries in English and Dutch ISBN 978‐90‐8585‐492‐0 C’est une prairie au petit jour, quelque part sur la Terre. Caché sous cette prairie s’étend un monde démesuré, grand comme une planète. Les herbes folles s’y transforment en jungles impénétrables, les cailloux deviennent montagnes et le plus modeste trou d’eau prend les dimensions d’un océan. Nuridsany C & Pérennou M 1996. -
Evolution, Discovery, and Interpretations of Arthropod Mushroom Bodies Nicholas J
Downloaded from learnmem.cshlp.org on September 28, 2021 - Published by Cold Spring Harbor Laboratory Press Evolution, Discovery, and Interpretations of Arthropod Mushroom Bodies Nicholas J. Strausfeld,1,2,5 Lars Hansen,1 Yongsheng Li,1 Robert S. Gomez,1 and Kei Ito3,4 1Arizona Research Laboratories Division of Neurobiology University of Arizona Tucson, Arizona 85721 USA 2Department of Ecology and Evolutionary Biology University of Arizona Tucson, Arizona 85721 USA 3Yamamoto Behavior Genes Project ERATO (Exploratory Research for Advanced Technology) Japan Science and Technology Corporation (JST) at Mitsubishi Kasei Institute of Life Sciences 194 Machida-shi, Tokyo, Japan Abstract insect orders is an acquired character. An overview of the history of research on the Mushroom bodies are prominent mushroom bodies, as well as comparative neuropils found in annelids and in all and evolutionary considerations, provides a arthropod groups except crustaceans. First conceptual framework for discussing the explicitly identified in 1850, the mushroom roles of these neuropils. bodies differ in size and complexity between taxa, as well as between different castes of a Introduction single species of social insect. These Mushroom bodies are lobed neuropils that differences led some early biologists to comprise long and approximately parallel axons suggest that the mushroom bodies endow an originating from clusters of minute basophilic cells arthropod with intelligence or the ability to located dorsally in the most anterior neuromere of execute voluntary actions, as opposed to the central nervous system. Structures with these innate behaviors. Recent physiological morphological properties are found in many ma- studies and mutant analyses have led to rine annelids (e.g., scale worms, sabellid worms, divergent interpretations. -
Atlas Des Orthoptères Du Gard
Atlas des orthoptères du Gard Cartes de répartition des espèces au 05/10/2021 : Vous allez consulter les cartes de répartition des espèces du Gard, proposant le pointage des observations validées ou pas encore validées : de ce fait il y a certainement des erreurs qui seront corrigées au fil de l'avancée du projet. Les cartes présentent des points rouges et des poins bleus : les premiers sont les mentions antérieures à l'année 2010, les seconds sont les observations contemporaines (depuis 2010 inclus). Observateurs : Nous avons le plaisir de remercier les observateurs qui ont contribué à la connaissance des papillons du Gard. Certains ont partagé directement leurs observations dans l'Observatoire du Patrimoine Naturel du Gard, d'autres utilisent l'application Observado développée par nos collègues des Pays-Bas, et un troisième lot provient d'échanges de données avec le projet d'Atlas des papillons de jour et libellules du Languedoc Roussillon, jusqu'à 2014 inclus. AUBIN gabrielle, Ador Quentin, Alaimo Jeanne, Albisser Léo, Alias Jean-Marie, Altertour , Andrieu Frédéric, Anonyme , Arnassant Stephan, Augustyniak Michel, Baldi Philippe, Bas Yves, Bastide Luc, Bauduin Julien, Beauval Cédric, Beils Antoine, Bence Stéphane, Bergue Emilie, Bernard Cyrielle, Bernier Christophe, Bernier Hanae, Bernier Lilou, Bernier Siméon, Bernon Jean-Marc, Berthelot Stéphane, Berthet François, Bertozzi Hervé, Bertozzi Maryvonne, Bertrand Justine, Beusse Bruno, Bidot Sylvain, Bigot Louis, Bizet Daniel, Blondel Françoise, Borel Nicolas, Bourrié Fanny, Branchu Sylvain,