Analysis of W± Bosons with ALICE: Effect of Alignment on W± Bosons Analysis
Total Page:16
File Type:pdf, Size:1020Kb
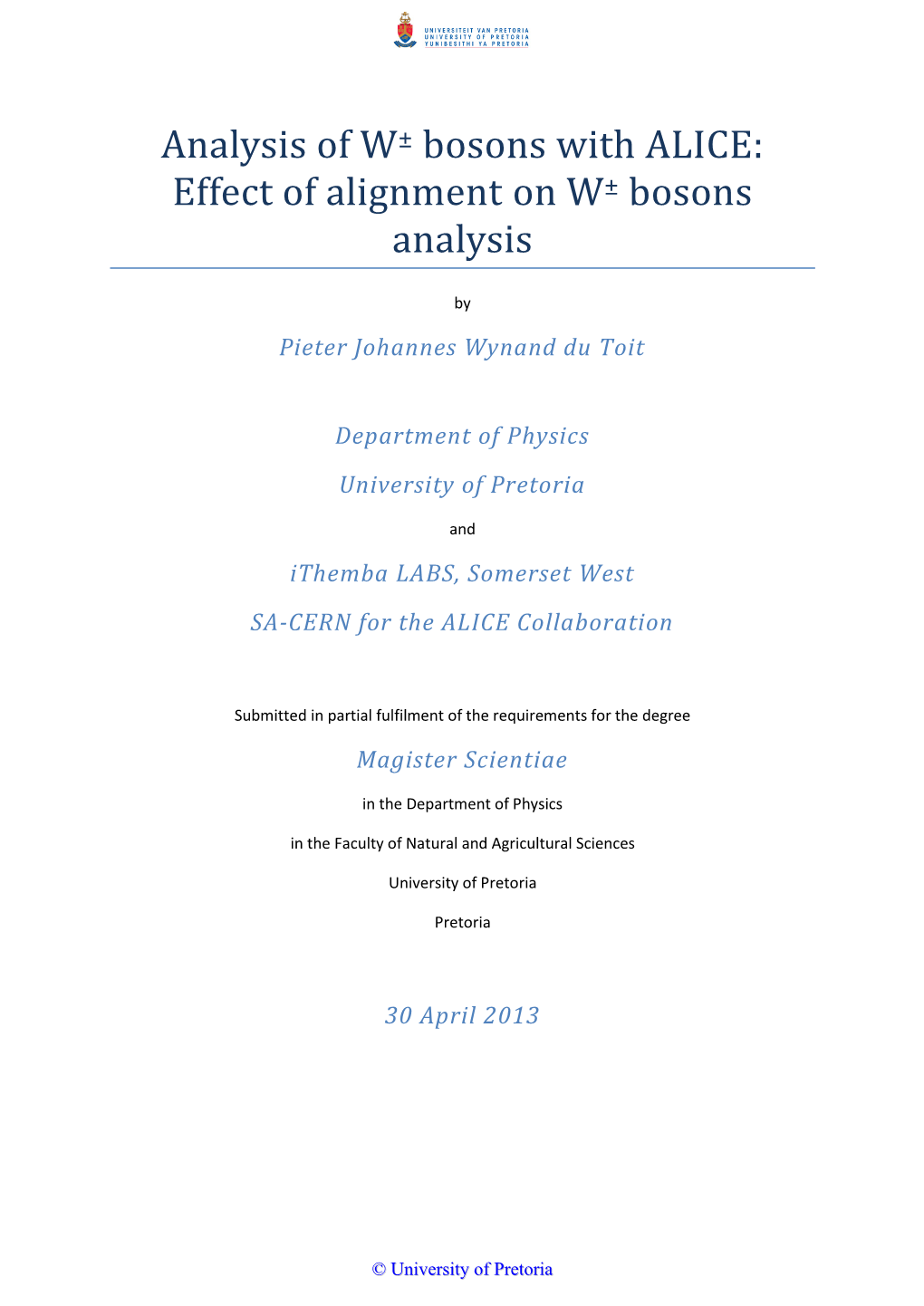
Load more
Recommended publications
-
NA61/SHINE Facility at the CERN SPS: Beams and Detector System
Preprint typeset in JINST style - HYPER VERSION NA61/SHINE facility at the CERN SPS: beams and detector system N. Abgrall11, O. Andreeva16, A. Aduszkiewicz23, Y. Ali6, T. Anticic26, N. Antoniou1, B. Baatar7, F. Bay27, A. Blondel11, J. Blumer13, M. Bogomilov19, M. Bogusz24, A. Bravar11, J. Brzychczyk6, S. A. Bunyatov7, P. Christakoglou1, T. Czopowicz24, N. Davis1, S. Debieux11, H. Dembinski13, F. Diakonos1, S. Di Luise27, W. Dominik23, T. Drozhzhova20 J. Dumarchez18, K. Dynowski24, R. Engel13, I. Efthymiopoulos10, A. Ereditato4, A. Fabich10, G. A. Feofilov20, Z. Fodor5, A. Fulop5, M. Ga´zdzicki9;15, M. Golubeva16, K. Grebieszkow24, A. Grzeszczuk14, F. Guber16, A. Haesler11, T. Hasegawa21, M. Hierholzer4, R. Idczak25, S. Igolkin20, A. Ivashkin16, D. Jokovic2, K. Kadija26, A. Kapoyannis1, E. Kaptur14, D. Kielczewska23, M. Kirejczyk23, J. Kisiel14, T. Kiss5, S. Kleinfelder12, T. Kobayashi21, V. I. Kolesnikov7, D. Kolev19, V. P. Kondratiev20, A. Korzenev11, P. Koversarski25, S. Kowalski14, A. Krasnoperov7, A. Kurepin16, D. Larsen6, A. Laszlo5, V. V. Lyubushkin7, M. Mackowiak-Pawłowska´ 9, Z. Majka6, B. Maksiak24, A. I. Malakhov7, D. Maletic2, D. Manglunki10, D. Manic2, A. Marchionni27, A. Marcinek6, V. Marin16, K. Marton5, H.-J.Mathes13, T. Matulewicz23, V. Matveev7;16, G. L. Melkumov7, M. Messina4, St. Mrówczynski´ 15, S. Murphy11, T. Nakadaira21, M. Nirkko4, K. Nishikawa21, T. Palczewski22, G. Palla5, A. D. Panagiotou1, T. Paul17, W. Peryt24;∗, O. Petukhov16 C.Pistillo4 R. Płaneta6, J. Pluta24, B. A. Popov7;18, M. Posiadala23, S. Puławski14, J. Puzovic2, W. Rauch8, M. Ravonel11, A. Redij4, R. Renfordt9, E. Richter-Wa¸s6, A. Robert18, D. Röhrich3, E. Rondio22, B. Rossi4, M. Roth13, A. Rubbia27, A. Rustamov9, M. -
The NA61/SHINE Experiment: Beams and Detector System
EUROPEAN LABORATORY FOR PARTICLE PHYSICS The NA61/SHINE experiment: beams and detector system By the NA61 Collaboration http://na61.web.cern.ch Abstract NA61/SHINE (SPS Heavy Ion and Neutrino Experiment) is a multi-purpose fixed target experiment to study hadron production in hadron-nucleus and nucleus-nucleus collisions at the CERN Super Proton Synchrotron. It recorded the first physics data with hadron beams in 2009 and with ion beams (secondary 7Be beams) in 2011. NA61/SHINE has greatly profited from the long development of the CERN pro- ton and ion sources and the accelerator chain as well as the H2 beam line of the CERN North Area. The latter has recently been modified to also serve as a fragment separator as needed to produce the Be beams for NA61/SHINE. Numerous compo- nents of the NA61/SHINE set-up were inherited from its predecessors, in particular, the last one, the NA49 experiment. Important new detectors and upgrades of the inherited equipment were introduced the NA61/SHINE Collaboration. This paper describes the NA61/SHINE experimental facility and used beams by the CERN Long Shutdown I, March 2013. June 11, 2013 Contents 1 Introduction 5 2 Beams 6 2.1 The proton acceleration chain . 6 2.2 The ion accelerator chain . 7 2.3 H2 beam line . 9 2.4 Hadron beams . 9 2.5 Primary and secondary ion beams . 10 3 Beam detectors and triggers 13 3.1 Beam counters . 13 3.2 A-detector . 14 3.3 Beam Position Detectors . 14 3.4 Z-detectors . 15 3.5 Trigger system . -
Work Project Report – Summer Student Programme 2018
Work Project Report – Summer Student Programme 2018 STUDENT: KATHARINA KOLATZKI MAIN SUPERVISOR: MICHA MOSKOVIC SECOND SUPERVISOR: ANNETTE HOLTKAMP Abstract With this report, I summarize the work conducted during my internship with the CERN Scientific Information Service as part of the Summer Student Programme 2017. I was in charge of writing Wikipedia articles about CERN experiments, accelerators and scientists. In total, I have contributed seven articles to the English Wikipedia and four to the German Wikipedia, next to smaller improvements on other articles and platforms. Introduction It is one of CERN’s main goals not just to conduct fundamental research in the field of high-energy physics, but also to share its vision and discoveries with society. Therefore, a good communication strategy is of key interest in this endeavour. CERN’s Scientific Information Service (SIS) manages the library as well as the historical and scientific archives of CERN, ensuring that both scientists and the general public have convenient access to the contents produced at CERN. The distribution of this knowledge can be implemented via different methods and media. Next to the physical archives and library, online databases have grown more and more important in recent years. These are for example the CERN Grey Book, the high-energy physics information platform INSPIRE, the CERN Document Server CDS and the free encyclopedia Wikipedia. During my Summer Student Internship between 25 June 2018 and 17 August 2018, I had the task to improve the representation of past CERN accelerators, experiments and important scientists on Wikipedia. In total, I have submitted 129 edits to the English Wikipedia. -
Sample-Efficient Reinforcement Learning for CERN Accelerator Control
PHYSICAL REVIEW ACCELERATORS AND BEAMS 23, 124801 (2020) Review Article Sample-efficient reinforcement learning for CERN accelerator control Verena Kain ,* Simon Hirlander, Brennan Goddard , Francesco Maria Velotti , and Giovanni Zevi Della Porta CERN, 1211 Geneva 23, Switzerland Niky Bruchon University of Trieste, Piazzale Europa, 1, 34127 Trieste TS, Italy Gianluca Valentino University of Malta, Msida, MSD 2080, Malta (Received 7 July 2020; accepted 9 November 2020; published 1 December 2020) Numerical optimization algorithms are already established tools to increase and stabilize the performance of particle accelerators. These algorithms have many advantages, are available out of the box, and can be adapted to a wide range of optimization problems in accelerator operation. The next boost in efficiency is expected to come from reinforcement learning algorithms that learn the optimal policy for a certain control problem and hence, once trained, can do without the time-consuming exploration phase needed for numerical optimizers. To investigate this approach, continuous model-free reinforcement learning with up to 16 degrees of freedom was developed and successfully tested at various facilities at CERN. The approach and algorithms used are discussed and the results obtained for trajectory steering at the AWAKE electron line and LINAC4 are presented. The necessary next steps, such as uncertainty aware model-based approaches, and the potential for future applications at particle accelerators are addressed. DOI: 10.1103/PhysRevAccelBeams.23.124801 I. INTRODUCTION AND MOTIVATION comprises about 1700 magnet power supplies alone, can be exploited efficiently. The CERN accelerator complex consists of various There are still many processes at CERN’s accelerators, normal conducting as well as super-conducting linear however, that require additional control functionality. -
Advanced Proton Driven Plasma Wakefield Acceleration Experiment at CERN
AWAKE, the Advanced Proton Driven Plasma Wakefield Acceleration Experiment at CERN Edda Gschwendtner, CERN for the AWAKE Collaboration Outline • Motivation • Plasma Wakefield Acceleration • AWAKE • Outlook 2 Motivation: Increase Particle Energies • Increasing particle energies probe smaller and smaller scales of matter • 1910: Rutherford: scattering of MeV scale alpha particles revealed structure of atom • 1950ies: scattering of GeV scale electron revealed finite size of proton and neutron • Early 1970ies: scattering of tens of GeV electrons revealed internal structure of proton/neutron, ie quarks. • Increasing energies makes particles of larger and larger mass accessible • GeV type masses in 1950ies, 60ies (Antiproton, Omega, hadron resonances… • Up to 10 GeV in 1970ies (J/Psi, Ypsilon…) • Up to ~100 GeV since 1980ies (W, Z, top, Higgs…) • Increasing particle energies probe earlier times in the evolution of the universe. • Temperatures at early universe were at levels of energies that are achieved by particle accelerators today • Understand the origin of the universe • Discoveries went hand in hand with theoretical understanding of underlying laws of nature Standard Model of particle physics 3 Motivation: High Energy Accelerators • Large list of unsolved problems: • What is dark matter made of? What is the reason for the baryon-asymmetry in the universe? What is the nature of the cosmological constant? … • Need particle accelerators with new energy frontier 30’000 accelerators worldwide! Also application of accelerators outside particle physics in medicine, material science, biology, etc… 4 LHC Large Hadron Collider, LHC, 27 km circumference, 7 TeV 5 Circular Collider Electron/positron colliders: limited by synchrotron radiation Hadron colliders: limited by magnet strength FCC, Future Circular Collider 80 – 100 km diameter Electron/positron colliders: 350 GeV Hadron (pp) collider: 100 TeV 20 T dipole magnets. -
STFC , Accelerator Review Panel Report
Accelerator Review Report 2014 Table of Contents 1. Executive Summary ....................................................................................................... 2 2. Background .................................................................................................................... 3 3. Review Process ............................................................................................................. 3 3.1 Review Panel .......................................................................................................... 4 3.2 Meetings of the panel .............................................................................................. 4 3.3 Areas of review discussions .................................................................................... 4 3.4 Information gathering .............................................................................................. 5 4. Review ........................................................................................................................... 5 4.1 Overview of the current accelerator programme ..................................................... 5 4.2 Governance ............................................................................................................ 7 4.3 Neutron Sources ................................................................................................... 11 4.4 Synchrotron Light Sources .................................................................................... 18 4.5 Free Electron Lasers -
LHC Season 2 Zation for Nuclear Research, on the Franco-Swiss Border Facts & Figures Near Geneva, Switzerland
The Large Hadron Collider (LHC) is the most powerful par- ticle accelerator ever built. The accelerator sits in a tunnel 100 metres underground at CERN, the European Organi- LHC Season 2 zation for Nuclear Research, on the Franco-Swiss border facts & figures near Geneva, Switzerland. CERN's Accelerator Complex WHAT IS THE LHC? CMS explained almost all experimental results in The LHC is a particle accelerator that pushes particle physics. But the Standard Model is LHC North Area incomplete. It leaves many questions open, protons or ions to near the speed of light. It 2008 (27 km) ALICE TT20 LHCb which the LHC will help to answer. consists of a 27-kilometre ring of supercon- TT40 TT41 SPS 1976 (7 km) TI8 TI2 ducting magnets with a number of accelerating TT10 ATLAS AWAKE HiRadMat 2016 • What is the origin of mass? The Standard 2011 TT60 structures that boost the energy of the particles ELENA AD Model does not explain the origins of mass, nor TT2 2016 (31 m) 1999 (182 m) BOOSTER 1972 (157 m) why some particles are very heavy while others along the way. ISOLDE p 1989 p East Area have no mass at all. However, theorists Robert n-ToF PS 2001 H+ 1959 (628 m) Brout, François Englert and Peter Higgs made LINAC 2 CTF3 neutrons e– WHY IS IT CALLed the “Large HADRON LEIR LINAC 3 a proposal that was to solve this problem. The Ions 2005 (78 m) COLLider”? Brout-Englert-Higgs mechanism gives a mass p (proton) ion neutrons p– (antiproton) electron proton/antiproton conversion to particles when they interact with an invi- • "Large" refers to its size, approximately 27km • Inside the LHC, two particle beams travel at in circumference. -
Preparation of a Primary Argon Beam for the CERN Fixed Target Physics ICIS ‘13 D
Preparation of a Primary Argon Beam for the CERN Fixed Target Physics ICIS ‘13 D. Küchler, M. O’Neil, R. Scrivens, J. Sta ord-Haworth, CERN, BE/ABP/HSL, 1211 Geneva 23, Switzerland R. W. Thomae, iThemba LABS, P.O. Box 722, Somerset West 7130, South Africa Abstract SOURCE AND LINAC SOURCE AND LINAC OPEN ISSUES II PERFORMANCE PERFORMANCE II he fixed target experiment NA61 in the North Area n the bias disk metal flakes had been accumulated of the SPS is studying phase transitions in strongly fter the spectrometer in the Faraday cup FC2 which could be identified as tantalum, the material T he operation of the source was quite simple compared O interacting matter. Up to now they used the primary currents in the order 120-140 eµA were measured. the bias disk is made of. The amount of sputtered material to the usual lead operation. The source was fed from A beams available from the CERN accelerator complex T The transformer in front of the RFQ showed 95-115 eµA. after only 10 weeks is of concern because the set-up of an argon bottle with a feedback-controlled needle valve. (protons and lead ions) or fragmented beams created In the Faraday cup after the RFQ 70-90 eµA and at the the accelerator chain and the experiment itself will take As feedback signal the pressure downstream of the valve from the primary lead ion beam. end of the linac in the transformer TRA15 55-65 eµA were around 8 months, so it is foreseen to change the plasma was used. -
CERN-THESIS-2012-336 University of Trieste
CERN-THESIS-2012-336 University of Trieste Faculty of Mathematical, Physical and Natural Sciences Master of Science in Physics Measurement of the associated production of a Z boson and hadronic jets with the CMS detector at LHC Candidate: Supervisor: Tomo Umer Dr. Giuseppe Della Ricca Assistant supervisor: Dr. Fabio Cossutti Academic Year 2011/2012 - Summer Session . To my best friend and my girlfriend, who are luckily the same person. Contents Index i Introduzione 1 Introduction 3 1 The Physics of LHC and the CMS detector 5 1.1 Large Hadron Collider . .5 1.1.1 A run-down of the accelerator . .7 1.1.2 Current LHC operational conditions . .8 1.1.3 Coordinate system and kinematic variables . 10 1.2 The Compact Muon Solenoid . 13 1.2.1 Physics goals . 13 1.2.2 Overview of the CMS detector . 15 1.2.3 Tracker . 17 1.2.4 Electromagnetic calorimeter . 20 1.2.5 Hadronic calorimeter . 24 1.2.6 Superconducting solenoidal magnet . 25 1.2.7 Muon detectors . 26 1.2.8 Trigger and Data acquisition system . 28 2 Theoretical basis for the Z + jets production 31 2.1 Basis of the Standard Model . 31 2.1.1 Electroweak interactions . 34 2.1.2 Strong interactions . 35 2.1.3 Description of a proton-proton collision . 36 2.2 Z + jets associated production . 37 2.2.1 Drell-Yan process . 37 2.2.2 Multijet production . 40 2.2.3 Study of the associated production of Z boson + jets at the LHC . 41 2.3 Jets . 42 3 Monte Carlo Event Generators 45 3.1 Introduction to Event Generators . -
LHC-Project-Report-799 CHARACTERISATION AND
EUROPEAN ORGANIZATION FOR NUCLEAR RESEARCH European Laboratory for Particle Physics LHC-Project-Report-799 Large Hadron Collider Project CHARACTERISATION AND PERFORMANCE OF THE CERN ECR4 ION SOURCE C. Andresen, J. Chamings, V. Coco, C.E. Hill, D. Kuchler, A. Lombardi, E. Sargsyan and R. Scrivens Abstract To optimise the heavy ion injector for the LHC, a good knowledge of the parameters of the ECR4 ion source and the beam transport in the Low Energy Beam Transport (LEBT) for a lead ion beam is necessary. Results of the emittance measurements of the full beam (O + Pb) leaving the source using a scanning slit and profile monitor will be presented. Furthermore, the emittance of a single charge state after the source has been measured using a solenoid scan coupled to a guillotine and spectrometer. The results for last year’s operation for the SPS fixed target physics with indium will also be presented. Paper Presented at the 16th International Workshop on ECR Ion Sources, ECRIS'04, September 26-30 2004, Berkeley, CA Geneva, Switzerland 16 November, 2004 Characterisation And Performance Of The CERN ECR4 Ion Source C. Andresen, J. Chamings, V. Coco, C.E. Hill, D. Kuchler, A. Lombardi, E. Sargsyan and R. Scrivens AB Department, CERN, 1211 Geneva 23, Switzerland Abstract. To optimise the heavy ion injector for the LHC, a good knowledge of the parameters of the ECR4 ion source and the beam transport in the Low Energy Beam Transport (LEBT) for a lead ion beam is necessary. Results of the emittance measurements of the full beam (O + Pb) leaving the source using a scanning slit and profile monitor will be presented. -
Delft University of Technology Precision Improvement in Optical
Delft University of Technology MSc. Thesis Precision Improvement in Optical Alignment Systems of Linear Colliders Pre-aligning the proposed Compact Linear Collider using Rasnik Systems Supervisors: Dr. Ir. H. van der Graaf Prof. Dr. Ir. T.M. Klapwijk Author: Joris van Heijningen February 12, 2012 Delft University of Technology MSc. Thesis Precision Improvement in Optical Alignment Systems of Linear Colliders Pre-aligning the proposed Compact Linear Collider using Rasnik Systems Supervisors: Dr. Ir. H. van der Graaf Prof. Dr. Ir. T.M. Klapwijk Author: Joris van Heijningen The research described in this thesis was performed in the Detector Research and Development group of the Dutch National Institute for Subatomic Physics (Nikhef), Science Park 105, 1098 XJ Amsterdam, The Netherlands, and in the Quantum Nanoscience department of the Delft University of Technology, Lorentzweg 1, 2628 CJ Delft, The Netherlands, in collaboration with the Accelerator & Beam Physics Survey and Align- ment group (ABP-SU) of the Beams Department (BE) at the European Organisation for Nuclear Research (CERN), CH-1211 Gen`eve 23, Switzerland Abstract Still to be written Contents 1 Introduction 3 1.1 Context and motivation . .3 1.2 Outline . .4 2 Linear Colliders 5 2.1 Operation of linear accelerators . .6 2.2 Present linear accelerators . .9 2.3 Linear collider alignment . 10 2.3.1 The Large Rectangular Fresnel Lens System . 10 2.3.2 Poisson Alignment Reference System . 11 2.3.3 Wire Positioning System . 12 2.3.4 Hydrostatic Level System . 14 2.4 The ILC and the CLiC . 14 3 Preferred alignment system 18 3.1 Rasnik . -
DOCTORAL THESIS June 2010 David Belohrad
Czech Technical University in Prague Faculty of Electrical Engineering Department of Measurement DOCTORAL THESIS CERN-THESIS-2010-131 05/10/2010 June 2010 David Bˇelohrad Czech Technical University in Prague Faculty of Electrical Engineering Department of Measurement Fast Beam Intensity Measurements for the LHC Doctoral Thesis David Bˇelohrad Geneva, April 2010 Ph.D. Programme: Electrical Engineering and Information Technology Branch of study: Measurement and Instrumentation Supervisor: doc. Ing. Petr Kaˇspar,CSc. DEDICATION Writing this thesis was a long term competition with myself. Competition full of slow and painful learning, searching for correct answers, and frustration in times when the inspiration betrayed me. The winners are however those who sustain. I want to dedicate this thesis to my wife, Barbora, without whom this thesis might not have been written, and to whom I am greatly indebted. You have been with me all the distressful way through, seamlessly taking care of our family affairs, and providing me with all those essential little things which allowed me to finish the task. Thank you for your love, support, and source of encouragement and inspiration that you have always given me. Thank you as well for your insistence, which boosted me in the rainy days. I want to dedicate this thesis to my son Erik as well. Thank you for your patience. I promise to spend more time with you. I love you both 1 ACKNOWLEDGEMENT I want to sincerely thank to my supervisor, Assoc. Prof. Petr Kaˇspar, for his supreme guidance during my research and study. He was always accessible and willing to help me in research and in technical matters related to the university.