Structural and Mechanistic Insights Into the Biosynthesis of CDP-Archaeol in Membranes
Total Page:16
File Type:pdf, Size:1020Kb
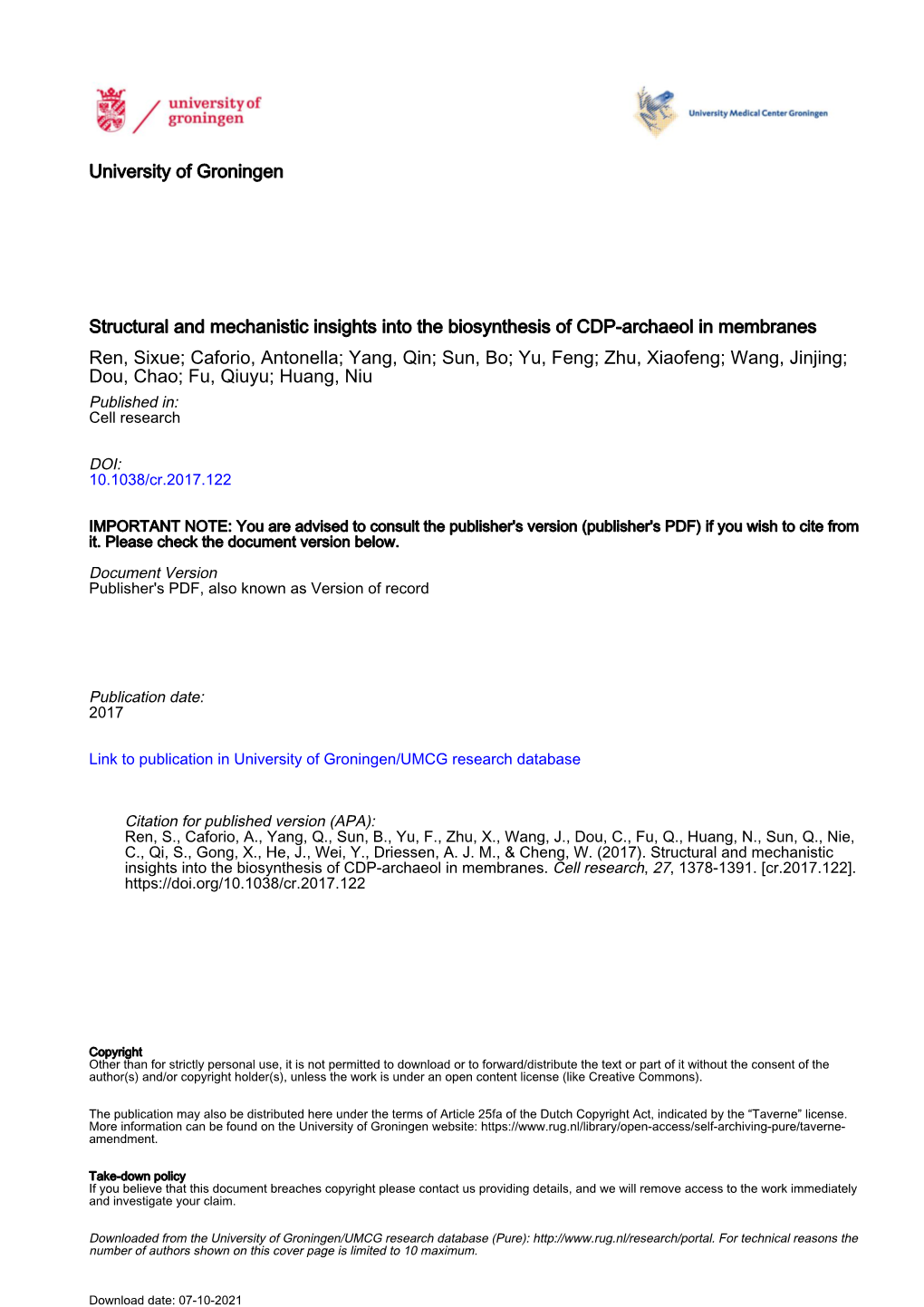
Load more
Recommended publications
-
The Pennsylvania State University the Graduate School Department
The Pennsylvania State University The Graduate School Department of Geosciences MOLECULAR AND ISOTOPIC INVESTIGATIONS OF THE BIOGEOCHEMISTRY OF ARCHAEAL ETHER LIPIDS A Thesis in Geosciences by Courtney Hanna Turich © 2006 Courtney Hanna Turich Submitted in Partial Fulfillment of the Requirements for the Degree of Doctor of Philosophy December 2006 ii The thesis of Courtney Hanna Turich was reviewed and approved* by the following: Katherine H. Freeman Professor of Geosciences Graduate Program Chair Thesis Advisor Chair of Committee Mary Ann Bruns Associate Professor of Crop and Soil Sciences Michael A. Arthur Professor of Geosciences Christopher House Associate Professor of Geosciences A. Daniel Jones Professor of Chemistry *Signatures are on file in the Graduate School iii ABSTRACT Once thought to inhabit only extreme environments, the Archaea are now known to occur globally in oceans, marshes, lakes, sediments, and soils. Archaea abundance and metabolic diversity link these microbes to important biogeochemical transformations. Archaea also generate abundant and diagnostic membrane lipids that are widespread in modern environments as well as the sedimentary record. These lipid compounds offer important evidence for past Archaea distribution and activity, and are a key means for understanding archaeal contributions to biogeochemical cycles, especially carbon and nitrogen, over Earth’s history. To link records of naturally occurring lipid to their biological and metabolic origins, I studied archaeal lipid distributions from a global set of modern waters in order to test the hypothesis that specific lipid assemblages will correspond to specific genotypic groups. Cluster analysis showed that marine lipid distribution patterns fell into two groups: the epipelagic zone and mesopelagic/upwelling zones. -
Lipid Analysis of CO2-Rich Subsurface Aquifers Suggests an Autotrophy-Based Deep Biosphere with Lysolipids Enriched in CPR Bacteria
The ISME Journal (2020) 14:1547–1560 https://doi.org/10.1038/s41396-020-0624-4 ARTICLE Lipid analysis of CO2-rich subsurface aquifers suggests an autotrophy-based deep biosphere with lysolipids enriched in CPR bacteria 1,2 3,4 1,3 3 3 Alexander J. Probst ● Felix J. Elling ● Cindy J. Castelle ● Qingzeng Zhu ● Marcus Elvert ● 5,6 6 1 7,9 7 Giovanni Birarda ● Hoi-Ying N. Holman ● Katherine R. Lane ● Bethany Ladd ● M. Cathryn Ryan ● 8 3 1 Tanja Woyke ● Kai-Uwe Hinrichs ● Jillian F. Banfield Received: 20 November 2018 / Revised: 5 February 2020 / Accepted: 25 February 2020 / Published online: 13 March 2020 © The Author(s) 2020. This article is published with open access Abstract Sediment-hosted CO2-rich aquifers deep below the Colorado Plateau (USA) contain a remarkable diversity of uncultivated microorganisms, including Candidate Phyla Radiation (CPR) bacteria that are putative symbionts unable to synthesize membrane lipids. The origin of organic carbon in these ecosystems is unknown and the source of CPR membrane lipids remains elusive. We collected cells from deep groundwater brought to the surface by eruptions of Crystal Geyser, sequenced 1234567890();,: 1234567890();,: the community, and analyzed the whole community lipidome over time. Characteristic stable carbon isotopic compositions of microbial lipids suggest that bacterial and archaeal CO2 fixation ongoing in the deep subsurface provides organic carbon for the complex communities that reside there. Coupled lipidomic-metagenomic analysis indicates that CPR bacteria lack complete lipid biosynthesis pathways but still possess regular lipid membranes. These lipids may therefore originate from other community members, which also adapt to high in situ pressure by increasing fatty acid unsaturation. -
Interpreting Sources and Endocrine Active Components of Trace Organic Contaminant Mixtures in Minnesota Lakes
INTERPRETING SOURCES AND ENDOCRINE ACTIVE COMPONENTS OF TRACE ORGANIC CONTAMINANT MIXTURES IN MINNESOTA LAKES by Meaghan E. Guyader © Copyright by Meaghan E. Guyader, 2018 All Rights Reserved A thesis submitted to the Faculty and the Board of Trustees of the Colorado School of Mines in partial fulfillment of the requirements for the degree of Doctor of Philosophy (Civil and Environmental Engineering). Golden, Colorado Date _____________________________ Signed: _____________________________ Meaghan E. Guyader Signed: _____________________________ Dr. Christopher P. Higgins Thesis Advisor Golden, Colorado Date _____________________________ Signed: _____________________________ Dr. Terri S. Hogue Professor and Department Head Department of Civil and Environmental Engineering ii ABSTRACT On-site wastewater treatment systems (OWTSs) are a suspected source of widespread trace organic contaminant (TOrC) occurrence in Minnesota lakes. TOrCs are a diverse set of synthetic and natural chemicals regularly used as cleaning agents, personal care products, medicinal substances, herbicides and pesticides, and foods or flavorings. Wastewater streams are known to concentrate TOrC discharges to the environment, particularly accumulating these chemicals at outfalls from centralized wastewater treatment plants. Fish inhabiting these effluent dominated environments are also known to display intersex qualities. Concurrent evidence of this phenomenon, known as endocrine disruption, in Minnesota lake fish drives hypotheses that OWTSs, the primary form of wastewater treatment in shoreline residences, may contribute to TOrC occurrence and the endocrine activity in these water bodies. The causative agents specific to fish in this region remain poorly understood. The objective of this dissertation was to investigate OWTSs as sources of TOrCs in Minnesota lakes, and TOrCs as potential causative agents for endocrine disruption in resident fish. -
Author's Response
GEORG–AUGUST-UNIVERSITY OF GOETTINGEN Geoscience Centre Department of Geobiology GZG Reinhardt, Manuel Goldschmidtstr. 3 Univ.Göttingen▪ GZG▪Abt.Geobiologie▪Goldschmidtstr.3▪37077 Göttingen▪Germany 37077 Göttingen Germany Phone: +49(0)551-39 13756 E-mail: [email protected] http://www. geobiologie.uni-goettingen.de Associate editor Biogeosciences Dr. Marcel van der Meer Göttingen, 04/18/2019 Submission of the revised manuscript bg-2018-513 Dear Marcel van der Meer, Also on behalf of my co-authors I would like to thank you, Jan W. de Leeuw, and the two anonymous reviewers for the thoughtful comments. As requested, we included the previously suggested corrections into the manuscript (see replies to RC1– 3, March 9th). In order to help you to track our changes and modifications, we attached the following documents: (i) Reply to your comments (ii) Replies to all reviewer comments (RC1–3 from March 9th) (iii) Tracked changes version of the manuscript (iv) Tracked changes version of the supplement We trust that the revised manuscript will meet the requirements of you and the reviewers. Yours sincerely, Manuel Reinhardt Comment from the editor: “I do think there is one issue you could spend a bit more time on explaining or clarifying. You use the Pleistocene settings as analogs for Archean hydrothermal cherts and I think you need to spend a little bit more time on this to make it more than a way to “sell” your Pleistocene study. The analogy is comparable deposits, hydrothermal cherts, right? I think it is entirely valid to test your approach, methods and types of analysis on a more modern setting to see what works and what doesn’t, what type of information you get etc., before actually working on these really old deposits. -
Phylogenetics of Archaeal Lipids Amy Kelly 9/27/2006 Outline
Phylogenetics of Archaeal Lipids Amy Kelly 9/27/2006 Outline • Phlogenetics of Archaea • Phlogenetics of archaeal lipids • Papers Phyla • Two? main phyla – Euryarchaeota • Methanogens • Extreme halophiles • Extreme thermophiles • Sulfate-reducing – Crenarchaeota • Extreme thermophiles – Korarchaeota? • Hyperthermophiles • indicated only by environmental DNA sequences – Nanoarchaeum? • N. equitans a fast evolving euryarchaeal lineage, not novel, early diverging archaeal phylum – Ancient archael group? • In deepest brances of Crenarchaea? Euryarchaea? Archaeal Lipids • Methanogens – Di- and tetra-ethers of glycerol and isoprenoid alcohols – Core mostly archaeol or caldarchaeol – Core sometimes sn-2- or Images removed due to sn-3-hydroxyarchaeol or copyright considerations. macrocyclic archaeol –PMI • Halophiles – Similar to methanogens – Exclusively synthesize bacterioruberin • Marine Crenarchaea Depositional Archaeal Lipids Biological Origin Environment Crocetane methanotrophs? methane seeps? methanogens, PMI (2,6,10,15,19-pentamethylicosane) methanotrophs hypersaline, anoxic Squalane hypersaline? C31-C40 head-to-head isoprenoids Smit & Mushegian • “Lost” enzymes of MVA pathway must exist – Phosphomevalonate kinase (PMK) – Diphosphomevalonate decarboxylase – Isopentenyl diphosphate isomerase (IPPI) Kaneda et al. 2001 Rohdich et al. 2001 Boucher et al. • Isoprenoid biosynthesis of archaea evolved through a combination of processes – Co-option of ancestral enzymes – Modification of enzymatic specificity – Orthologous and non-orthologous gene -
Assessing the Use of Archaeal Lipids As Marine Environmental Proxies
EA41CH14-Ingalls ARI 30 April 2013 15:56 Assessing the Use of Archaeal Lipids as Marine Environmental Proxies Ann Pearson1 and Anitra E. Ingalls2 1Department of Earth and Planetary Sciences, Harvard University, Cambridge, Massachusetts 02138; email: [email protected] 2School of Oceanography, University of Washington, Seattle, Washington 98195; email: [email protected] Annu. Rev. Earth Planet. Sci. 2013. 41:359–84 Keywords First published online as a Review in Advance on paleothermometry, lipid biomarkers, TEX86, Thaumarchaeota, March 7, 2013 Euryarchaeota, marine prokaryotes The Annual Review of Earth and Planetary Sciences is online at earth.annualreviews.org Abstract by Harvard University on 12/05/13. For personal use only. This article’s doi: Archaea are abundant in marine and terrestrial aquatic environments, sed- 10.1146/annurev-earth-050212-123947 iments, and soils. They inhabit at least an 85◦C temperature range from Copyright c 2013 by Annual Reviews. the polar ocean to hydrothermal springs. Many Archaea produce membrane All rights reserved lipids called glycerol dialkyl glycerol tetraethers (GDGTs). Experiments on Annu. Rev. Earth Planet. Sci. 2013.41:359-384. Downloaded from www.annualreviews.org pure and enrichment cultures as well as an empirical correlation for ma- rine sediments (the TEX86 index) together show positive relationships be- tween temperature and the number of cyclopentane or cyclohexane rings in GDGTs. The resulting TEX86 paleotemperature proxy has been applied across a wide range of geologic history and depositional settings. The ex- act relationship between TEX86 and temperature, however, remains poorly understood. Environmental systems and cultures have different temperature dependencies, and the ecological niche(s) of aquatic Archaea are still a sub- ject of active investigation. -
Open Thesis 17May2011.Pdf
The Pennsylvania State University The Graduate School Department of Geosciences BIOGEOCHEMISTRY OF ISOPRENOID PRODUCTION AND ANAEROBIC HYDROCARBON BIODEGRADATION A Dissertation in Geosciences by Katherine S. Dawson © 2011 Katherine S. Dawson Submitted in Partial Fulfillment of the Requirements for the Degree of Doctor of Philosophy August 2011 The dissertation of Katherine S. Dawson was reviewed and approved* by the following: Jennifer L. Macalady Assistant Professor of Geosciences Dissertation Co-Advisor Co-Chair of Committee Katherine H. Freeman Professor of Geosciences Dissertation Co-Advisor Co-Chair of Committee Christopher H. House Associate Professor of Geosciences John M. Regan Associate Professor of Environmental Engineering Chris J. Marone Professor of Geosciences Associate Head for Graduate Programs and Research in Geosciences *Signatures are on file in the Graduate School iii ABSTRACT This dissertation is an exploration of microbial isoprenoid production and destruction by anaerobic hydrocarbon biodegradation. Isoprenoids are methyl-branched hydrocarbons, and include biomarkers from all three domains of life such as archaeal lipids, hopanoids, and sterols. Isoprenoid production was examined through variation in the molecular structure of archaeal lipids across a hypersaline gradient (Chapter 5). This study identified unsaturated analogues of archaeol in four halophilic archaeal strains and revealed an increase in the percentage of unsaturated lipids with increasing salinity. Anaerobic isoprenoid biodegradation was examined through the enrichment of bacteria under anaerobic conditions utilizing pristane as a carbon source (Chapter 2). Further analysis of anaerobic degradation utilized 13C-labelled phytane as a stable isotope tracer (Chapter 3). In both cases, a microbial community dominated by denitrifying Beta- and Gammaproteobacteria was responsible for the degradation of pristane and phytane. -
Using Archaeol to Investigate the Location of Methanogens in the Ruminant Digestive Tract
Livestock Science 164 (2014) 39–45 Contents lists available at ScienceDirect Livestock Science journal homepage: www.elsevier.com/locate/livsci Using archaeol to investigate the location of methanogens in the ruminant digestive tract C.A. McCartney a,b,n, I.D. Bull b, R.J. Dewhurst a,1 a Teagasc, Animal and Grassland Research and Innovation Centre, Grange, Dunsany, Co. Meath, Ireland b School of Chemistry, University of Bristol, Cantock's Close, Bristol, BS8 1TS, UK article info abstract Article history: The quantification of archaeol, a methanogen membrane lipid, may provide an alternative Received 28 August 2013 method to estimate methanogen abundance. The focus of this study was to determine the Received in revised form location of methanogens in the ruminant digestive tract using this biomarker. Archaeol 13 February 2014 was quantified in samples obtained from four lactating cows with rumen cannulae that Accepted 24 February 2014 grazed on either white clover (WC) or perennial ryegrass (PRG) in a changeover design study with three 3-week periods. Faeces were collected over the final 5 d of each period Keywords: and total rumen contents (TRC) were obtained on the final 2 days (day 1: 9 am; day Archaeol 2: 3 pm). Solid-associated microbes (SAM) and liquid-associated microbes (LAM) were Faeces also isolated from the TRC. Concentrations of archaeol in the TRC showed a significant diet Methanogen by time interaction, which may be related to diurnal grazing patterns and different rumen Rumen conditions associated with PRG or WC diets. There was significantly more archaeol associated with SAM than LAM, which may reflect difficulties of methanogen proliferation in the liquid phase. -
Quantitative Analysis of Terpenic Compounds in Microsamples of Resins by Capillary Liquid Chromatography
molecules Article Quantitative Analysis of Terpenic Compounds in Microsamples of Resins by Capillary Liquid Chromatography H. D. Ponce-Rodríguez 1,2 , R. Herráez-Hernández 1,* , J. Verdú-Andrés 1,* and P. Campíns-Falcó 1 1 MINTOTA Research Group, Department of Analytical Chemistry, Faculty of Chemistry, University of Valencia, Dr Moliner 50, 46100 Burjassot, Valencia, Spain; [email protected] (H.D.P.-R.); [email protected] (P.C.-F.) 2 Department of Chemical Control, Faculty of Chemistry and Pharmacy, National Autonomous University of Honduras, Ciudad Universitaria, 11101 Tegucigalpa, Honduras * Correspondence: [email protected] (R.H.-H); [email protected] (J.V.-A) Academic Editors: Pavel B. Drasar, Vladimir A. Khripach and Maria Carla Marcotullio Received: 10 October 2019; Accepted: 8 November 2019; Published: 10 November 2019 Abstract: A method has been developed for the separation and quantification of terpenic compounds typically used as markers in the chemical characterization of resins based on capillary liquid chromatography coupled to UV detection. The sample treatment, separation and detection conditions have been optimized in order to analyze compounds of different polarities and volatilities in a single chromatographic run. The monoterpene limonene and the triterpenes lupeol, lupenone, β-amyrin, and α-amyrin have been selected as model compounds. The proposed method provides linear responses and precision (expressed as relative standard deviations) of 0.6% to 17%, within the 1 0.5–10.0 µg mL− concentration interval; the limits of detection (LODs) and quantification (LOQs) 1 1 were 0.1–0.25 µg mL− and 0.4–0.8 µg mL− , respectively. -
Genomic Analysis of Uncultured Microbes in Marine Sediments
University of Tennessee, Knoxville TRACE: Tennessee Research and Creative Exchange Doctoral Dissertations Graduate School 12-2017 Singled Out: Genomic analysis of uncultured microbes in marine sediments Jordan Toby Bird University of Tennessee, [email protected] Follow this and additional works at: https://trace.tennessee.edu/utk_graddiss Recommended Citation Bird, Jordan Toby, "Singled Out: Genomic analysis of uncultured microbes in marine sediments. " PhD diss., University of Tennessee, 2017. https://trace.tennessee.edu/utk_graddiss/4829 This Dissertation is brought to you for free and open access by the Graduate School at TRACE: Tennessee Research and Creative Exchange. It has been accepted for inclusion in Doctoral Dissertations by an authorized administrator of TRACE: Tennessee Research and Creative Exchange. For more information, please contact [email protected]. To the Graduate Council: I am submitting herewith a dissertation written by Jordan Toby Bird entitled "Singled Out: Genomic analysis of uncultured microbes in marine sediments." I have examined the final electronic copy of this dissertation for form and content and recommend that it be accepted in partial fulfillment of the equirr ements for the degree of Doctor of Philosophy, with a major in Microbiology. Karen G. Lloyd, Major Professor We have read this dissertation and recommend its acceptance: Mircea Podar, Andrew D. Steen, Erik R. Zinser Accepted for the Council: Dixie L. Thompson Vice Provost and Dean of the Graduate School (Original signatures are on file with official studentecor r ds.) Singled Out: Genomic analysis of uncultured microbes in marine sediments A Dissertation Presented for the Doctor of Philosophy Degree The University of Tennessee, Knoxville Jordan Toby Bird December 2017 Copyright © 2017 by Jordan Bird All rights reserved. -
Identification of CDP-Archaeol Synthase, a Missing Link of Ether Lipid Biosynthesis in Archaea
Chemistry & Biology Article Identification of CDP-Archaeol Synthase, a Missing Link of Ether Lipid Biosynthesis in Archaea Samta Jain,1,2,4 Antonella Caforio,1,2 Peter Fodran,3 Juke S. Lolkema,1,2 Adriaan J. Minnaard,3 and Arnold J.M. Driessen1,2,* 1Department of Molecular Microbiology, Groningen Biomolecular Sciences and Biotechnology Institute, University of Groningen, 9747 AG Groningen, the Netherlands 2Zernike Institute for Advanced Materials, University of Groningen, 9747 AG Groningen, the Netherlands 3Stratingh Institute for Chemistry, University of Groningen, Nijenborgh 7, 9747 AG Groningen, the Netherlands 4Present address: Department of Medicine, Section of Infectious Diseases, Boston University School of Medicine, Boston, MA 02118, USA *Correspondence: [email protected] http://dx.doi.org/10.1016/j.chembiol.2014.07.022 SUMMARY composition (Lombard et al., 2012a). Understanding the bio- synthetic pathways leading to the formation and regulation of Archaeal membrane lipid composition is distinct membrane lipid composition would help decipher the unknown from Bacteria and Eukarya, consisting of isoprenoid aspects of early evolution. Although ether phospholipids or fatty chains etherified to the glycerol carbons. Biosyn- acid based ether lipids are also found in a few bacteria and thesis of these lipids is poorly understood. Here we eukarya (Lorenzen et al., 2014), they differ from the isoprenoid identify and characterize the archaeal membrane derived archaeal ether lipids. The unique structure of archaeal protein CDP-archaeol synthase (CarS) that catalyzes membrane lipids is believed to be vital for the adaptation of these organisms to the extreme environmental conditions the transfer of the nucleotide to its specific archaeal (Koga and Morii, 2007; van de Vossenberg et al., 1998), but lipid substrate, leading to the formation of a CDP- this basic lipid architecture is found in all archaea including the activated precursor (CDP-archaeol) to which polar mesophilic Thaumarchaeota. -
Green and Sustainable Valorization of Bioactive Phenolic Compounds from Pinus By-Products
molecules Review Green and Sustainable Valorization of Bioactive Phenolic Compounds from Pinus By-Products Pedro Ferreira-Santos , Elisa Zanuso , Zlatina Genisheva, Cristina M. R. Rocha and José A. Teixeira * CEB—Centre of Biological Engineering, University of Minho, Campus de Gualtar, 4710-057 Braga, Portugal; [email protected] (P.F.-S.); [email protected] (E.Z.); [email protected] (Z.G.); [email protected] (C.M.R.R.) * Correspondence: [email protected]; Tel.: +253-604-406 Academic Editor: Vassiliki Oreopoulou Received: 2 June 2020; Accepted: 23 June 2020; Published: 25 June 2020 Abstract: In Europe, pine forests are one of the most extended forests formations, making pine residues and by-products an important source of compounds with high industrial interest as well as for bioenergy production. Moreover, the valorization of lumber industry residues is desirable from a circular economy perspective. Different extraction methods and solvents have been used, resulting in extracts with different constituents and consequently with different bioactivities. Recently, emerging and green technologies as ultrasounds, microwaves, supercritical fluids, pressurized liquids, and electric fields have appeared as promising tools for bioactive compounds extraction in alignment with the Green Chemistry principles. Pine extracts have attracted the researchers’ attention because of the positive bioproperties, such as anti-inflammatory, antimicrobial, anti-neurodegenerative, antitumoral, cardioprotective, etc., and potential industrial applications as functional foods, food additives as preservatives, nutraceuticals, pharmaceuticals, and cosmetics. Phenolic compounds are responsible for many of these bioactivities. However, there is not much information in the literature about the individual phenolic compounds of extracts from the pine species.