Crimson Team Final Report
Total Page:16
File Type:pdf, Size:1020Kb
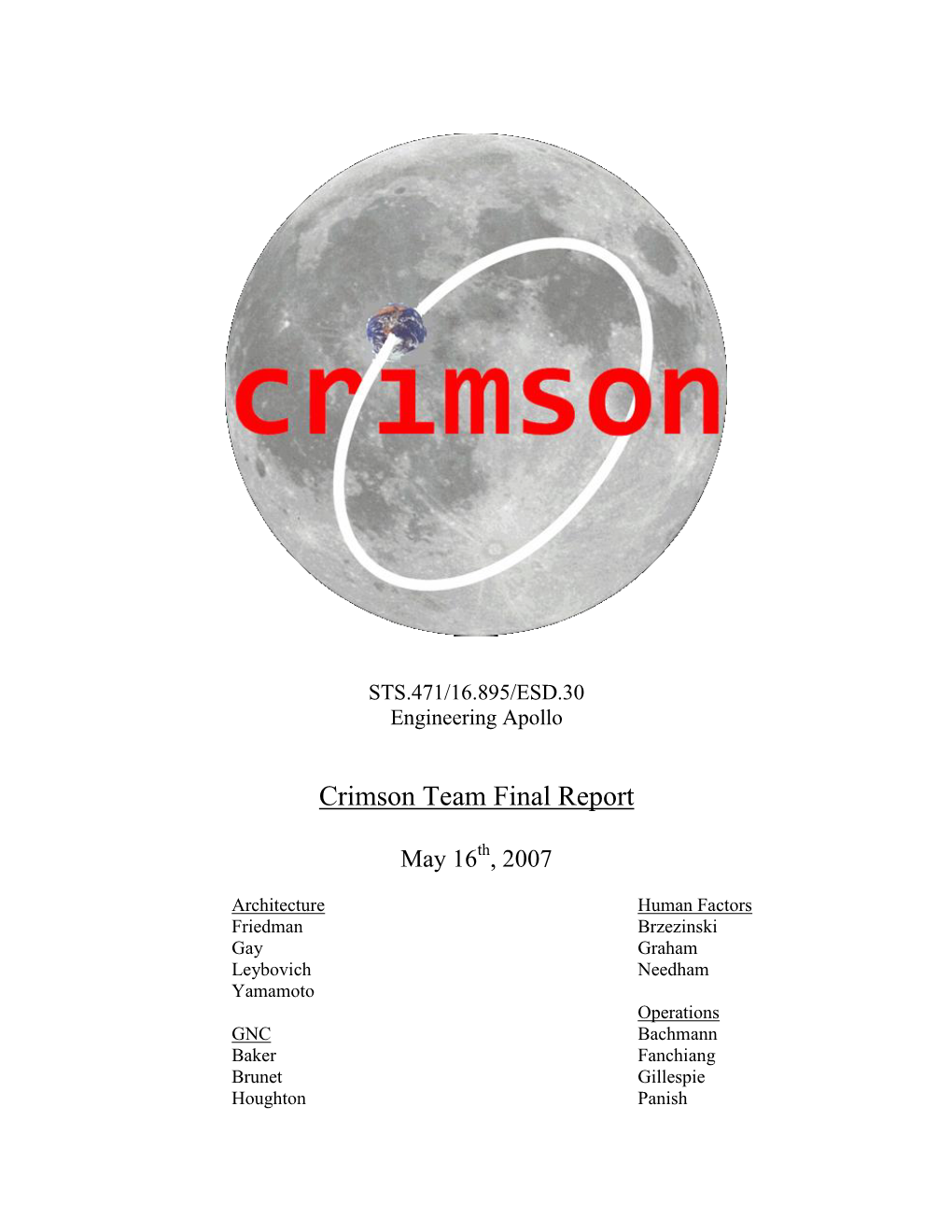
Load more
Recommended publications
-
THE DESCENT 2 Revisions by James Watkins
THE DESCENT 2 revisions by James Watkins 26th January 2008 Christian Colson Celador Films 39 Long Acre London WC2E 9LG Tel: +44 (0)207 8456988 EXT. APPALACHIAN MOUNTAINS - DUSK [HELICOPTER SHOTS] Storm clouds scud over a mountain range. Imposing peaks, impassable pine forest. A phone line RINGS OVER, CONNECTS to... VOICEMAIL MESSAGES. Ghost voices. SARAH CARTER (O.S.) Hi, this is Sarah Carter. I’m away for the rest of the month and won’t be picking up messages. BETH (O.S.) Hi, this is Beth. I’m in America, so please don’t leave me a message as it’s so expensive to pick them up! EXT. FOREST CLEARING - DUSK Two abandoned 4x4 jeeps. On one bumper, a ‘Rock chic’ sticker. Inside, a cellphone GLOWS on the dashboard. HOLLY (O.S.) Hey, this is Holly’s phone. No shit! Leave a fuckin’ message. Unless you’re Bobby Flynn- Bobby piss off you stalker creep. EXT. LOG CABIN - NIGHT A backwoods cabin. Dark forest encroaching all around. REBECCA (O.S.) Hi, this is Rebecca Van Ney. I’m out of the office until the 13th. In emergencies, you can reach me via Juno Kaplan on- INT. LOG CABIN - DUSK [STEADICAM] Eerily empty ROOMS, CORRIDORS. Party leftovers. The girls’ suitcases, clothes, cosmetics, photo albums, medication... A cellphone VIBRATES... JUNO KAPLAN (O.S.) This is Juno. I can’t make the phone right now. Please leave a short message and I’ll get back to you when I can. A long message BEEP. 2. INT. BOREHAM CAVERNS - DARKNESS Black screen. A MAN’S VOICE- MOUNTAIN RESCUE RANGER (O.S.) This is Pulaskie Mountain Rescue. -
Astro2020 APC White Paper Another Servicing Mission to Extend Hubble Space Telescope’S Science Past the Next Decade
Astro2020 APC White Paper Another Servicing Mission to Extend Hubble Space Telescope’s Science past the Next Decade Thematic Areas: Activity Project State of the Profession Principal Author: Name: Mercedes López-Morales Institution: Center for Astrophysics | Harvard & Smithsonian Email: [email protected] Phone: +1.617.496.7818 Co-authors: Kevin France (University of Colorado), Francesco R. Ferraro (University of Bologna), Rupali Chandar (University of Toledo), Steven Finkelstein (University of Texas at Austin), Stephane Charlot (Institut d’Astrophysique de Paris - CNRS/Sorbonne Université), Gilda Ballester (University of Arizona), Melina. C. Bersten (Instituto de Astrofísica de La Plata CONICET-UNLP; University of Tokyo), Jose M. Diego (Instituto de Fisica de Cantabria), Gastón Folatelli (Instituto de Astrofísica de La Plata CONICET-UNLP; University of Tokyo), Domingo García-Senz (Universitat Politécnica de Catalunya), Mauro Giavalisco (University of Massachusetts), Rolf A. Jansen (Arizona State University), Patrick L. Kelly (University of Minnesota), Thomas Maccarone (Texas Tech University), Seth Redfield (Wesleyan University), Pilar Ruiz-Lapuente (University of Barcelona), Steve Shore (University of Pisa), Nitya Kallivayalil (University of Virginia) Co-signers: Munazza K. Alam (Center for Astrophysics | Harvard & Smithsonian), Juan Manuel Alcalá (INAF – AO of Naples), Jay Anderson (Space Telescope Space Institute), Daniel Angerhausen (CSH, Bern University), Francesca Annibali (INAF – OAS of Bologna), Dániel Apai (University of Arizona), David Ardila (Jet Propulsion Laboratory), Santiago Arribas (Centro de Astrobiología CSIC-INTA), Hakim Atek (Institut d’astrophysique de Paris - CNRS/Sorbonne Université), Thomas R. Ayres, (University of Colorado), Francesca Bacciotti (INAF – OA of Florence), Beatriz Barbuy (Universidade de Sao Paulo), Joanna K. Barstow (University College London), Nate Bastian (Liverpool John Moores University), Natasha E. -
The Breeding Birds of Central Lower California (With Eleven Ills.)
20 Vol. xxx11 THE BREEDING BIRDS OF CENTRAL LOWER CALIFORNIA WITH ELEVEN ILLUSTRATIONS By GRIFFING BANCROFT A Contributionfrom the San Diego Societyof Natural History By wagon road and burro trail it is an even one hundred miles from Santa Rosalia, on the Gulf of California, to the tide line of the Pacific at San Ignacio Lagoon. The intervening country is essentially a desert. The summit, which is two thousand feet high in the passes and nearly three times that altitude in the mountains, lies within ten or twelve miles of the Gulf. The two water- sheds are thus of unequal length. They are also of quite distinct configuration. On the eastern side the descent to sea-level is abrupt and precipitous, checked by two rather extensive valleys. The long western slope, on the other hand, is broken by valleys, canons, and pretentious hills. It is marked with the weird formations which are characteristic of arid North America and which are here exaggerated. Angles and profiles of silhouetted hills and table-tops are unusually harsh and for- bidding. There is not even the softening effect of grandeur. Over the major portion of the entire region lava has flowed and mesa, valley and mountains are covered with dull brown rocks. This lava sheet, though of varying thickness, normally does not exceed three feet in depth. In overlaying the ancient sandstone it parallels the slopes of the hills and the sides of the canons, while on the mesas, and sometimes in the valleys too, it is as level as they and often stretches away as far as the eye can see. -
What Does It Mean to Be a Woman in Horror?
What Does it Mean to be a Woman in Horror? Presentation by Maya Hendl CONTENT WARNING This presentation contains descriptions of violence, torture, kidnapping, and murder. There are no explicit images of these acts taking place, but these acts are mentioned and (briefly) described. Table of Contents 1 2 Final Girls Female Antagonists Exploring examples of female villains, why they are Exploring what they & so scary, and their ability to are, why they exist, justify their motivations in and the contexts in comparison to male which they exist antagonists 1 Final Girls Exploring what they are, why they exist, and the contexts in which they exist What Does it Mean to be a “Final Girl”? The term “final girl” was coined by Carol J. Clover in her 1993 book Men, Women and Chain Saws: Gender in the Modern Horror Film. The “final girl” is the last woman or girl left alive in a horror movie, most often within the slasher genre, that must confront the killer. Clover describes final girls as typically being sexually unavailable at the start of the film, as having sex in a horror movie is a common trope that will most likely get you killed (Alternative Press Magazine). Clover goes on to describe how, once the final girl survives, she “is ‘purged’...of undesirable characteristics, such as pursuit of pleasure in her own right. An interesting feature of the genre is the ‘punishment’ of beauty and sexual availability” (Horror Fandom Wiki). Why Do Final Girls Exist? In horror films, women are more often than not left to fight the big bad all on their own. -
THE DESCENT 2005 (U.K.)
THE DESCENT 2005 (u.k.) Director Neil Marshall Producers Christian Colson, Paul Ritchie, Paul Smith Screenplay Neil Marshall Photography Sam McCurdy Music David Julyan Cast Shauna Macdonald, Natalie Mendoza, Alex Reid, Saskia Mulder, MyAnna Buring, Nora-Jane Noone, Oliver Milburn, Molly Kayll, Craig Conway, Leslie Simpson One would be hard-pressed to think of a horror film in recent years that has wowed both critics and audiences alike quite as much as Neil Marshall’s sophomore effort, The Descent. While his first film, Dog Soldiers (2002) was a fun riff on the werewolf movie, The Descent is almost humor-free and more than manages to deliver the goods. Recovering after a car accident in which she lost both her husband and young daughter, Sarah (Macdonald) gets back with her cohort of extreme sport-loving girlfriends for a spelunking expedition in the Appalachians. Juno (Mendoza), the reckless one of the pack, chooses an uncharted cave to explore, and the six friends go deep within the mountains. A cave-in cuts the The idea for the poster art women off from going back the way they came, and as they came from a venture forward they run into a race of “crawlers”—humanoid- photograph by like creatures with a taste for human flesh. Philippe Halsman Whereas Dog Soldiers was a jokey romp about masculinity, of Salvador Dalí, The Descent’s focus is on the women; not as traditional horror which was itself inspired by Dali’s movie women, weak and frightened by everything around gouache painting them, but as strong, capable and tough-as-nails chicks. -
An Animated Examination of Horror in Cinema
An Animated Examination of Cinematic Horror Elisa Stanis Spring 2019 Thesis submitted in completion of Honors Senior Capstone requirements for the DePaul University Honors Program Thesis Director: Devin Bell, Animation Faculty Reader: Brian Ferguson, Animation S t a n i s | 2 “The night is dark and full of terrors” - George R. R. Martin "What are common tropes in horror movies, and how have they evolved over time?" By researching the history of horror-related storytelling in cinema, I designed an animated short that pays homage to cinematic horror. My research explores the evolution of horror-related movies and alters the aesthetics of animation to fit differing visual themes. I delve into the details of designing a character that can be altered to exist in these conjoining sections, as well as how to best cinematically tell my story. Additionally, I specifically examine the role of young women in horror movies, as well as the idea of turning mundane actions into terrifying experiences. S t a n i s | 3 TABLE OF CONTENTS Abstract ________________________________________________________________________ 02 Acknowledgements ____________________________________________________________ 04 Introduction ____________________________________________________________________ 05 Concept to Creation ____________________________________________________________ 06 Designing a Protagonist _______________________________________________________ 08 Thematic Content: Structured by Segment: Traditional Horror (1960s) __________________________________________ -
FOX SEARCHLIGHT PICTURES Presents PATHÉ, BBC FILMS
FOX SEARCHLIGHT PICTURES Presents PATHÉ, BBC FILMS, INGENIOUS MEDIA and BFI present with the participation of CANAL+ and CINÉ+ A YORUBA SAXON / HARBINGER PICTURES / PERFECT WEEKEND / FILM UNITED PRODUCTION An AMMA ASANTE Film DAVID OYELOWO ROSAMUND PIKE JACK DAVENPORT TOM FELTON LAURA CARMICHAEL TERRY PHETO JESSICA OYELOWO ARNOLD OCENG NICHOLAS ROWE ANTON LESSER ANASTASIA HILLE JACK LOWDEN MERVEILLE LUKEBA and NICHOLAS LYNDHURST DIRECTED BY ........................................................................... AMMA ASANTE SCREENPLAY BY ..................................................................... GUY HIBBERT PRODUCED BY .......................................................................... RICK MCCALLUM ..................................................................................................... DAVID OYELOWO ..................................................................................................... PETER HESLOP ..................................................................................................... BRUNSON GREEN ..................................................................................................... JUSTIN MOORE-LEWY ..................................................................................................... CHARLIE MASON EXECUTIVE PRODUCERS ....................................................... CAMERON MCCRACKEN ..................................................................................................... CHRISTINE LANGAN .................................................................................................... -
1. Introducing the Splat Pack
1. INTRODUCING THE SPLAT PACK The ‘New Blood’: a Name Catches On Excitement about the Splat Pack seems to have been ignited with the April 2006 issue of the British film magazine Total Film. The issue featured an article by Alan Jones, entitled ‘The New Blood’. Its title was accentuated by a ‘Parental Advisory: Explicit Content’ label to let readers know they were about to enter forbidden, dangerous territory. Evidently, the local cineplex had been transformed into dangerous territory because ‘a host of bold new horror flicks’, such as Eli Roth’s Hostel and Alexandre Aja’s 2006 remake of Wes Craven’s 1977 shocker The Hills Have Eyes, had assaulted audiences with levels of brutality missing in ‘all those toothless remakes of Asian hits starring Jennifer Connelly, Naomi Watts and Sarah Michelle Gellar’ (Jones, 2006: 101, 102). Jones devotes his article to showcasing the young directors who were ‘taking back’ horror from purveyors of ‘watered-down’ genre movies (2006: 102). One of the auteurs in the movement featured in Jones’s article is Eli Roth who positions himself as one of the power players of this movement. Jones’s article features a photo of Roth brandishing a chainsaw, a devilish smirk on his face, surrounded by photos of bloody carnage from Roth’s Hostel, including an image of a man being castrated with a pair of bolt cutters. These grisly visuals imply that Roth can deliver the gory goods. In the article, Roth – a graduate of New York University’s film school whose father and mother are, respectively, a Harvard professor and fine artist – comes across as forcefully as these images 13 BERNARD 9780748685493 PRINT.indd 13 13/01/2014 10:43 Grahams HD:Users:Graham:Public:GRAHAM'S IMAC JOBS:14636 - EUP - BERNARD (TWC):BERNARD 9780748685493 PRINT SELLING THE SPLAT PACK would suggest. -
DAVID JOHNSTON +44 (0) 77927 63639 | [email protected]
DAVID JOHNSTON +44 (0) 77927 63639 | [email protected] FEATURE FILMS “Dark Shadows” Assisting colourist on providing colour managed rushes to Editorial on (Warner Brothers) site of shoot. Liasing with various departments (VFX, Camera, Production) to troubleshoot. Supplying graded VFX through pipeline to editorial. Workflow management “TT3D: Closer To The Edge” Online, stereo camera matching, client attended depth grade, 200+ (CinemaNX) stereo fixes “African Cats” DI consultant, HD conform, opticals, VFX, noise reduction (Disney Nature/Wild Horizons) “Where The Wild Things Are” 2k online, opticals, client attended reviews, support grading, pipeline (Warner Brothers/Village Roadshow) development “In The Loop” HD online conform (for film out), optical (BBC/Aramid) “The Boat That Rocked” multiple HD previews, online 2k conform, opticals, DI vfx (Working Title) “Nutcracker: The Untold Story” multiple 2k preview conform, client vfx sessions “Shanghai” 2k preview conform (The Weinstein Company) “The Chronicles Of Narnia: Prince Caspian” Pipeline development (Disney) TELEVISION “Frozen Planet” Conform, grade prep/assist, workflow consultancy (BBC) “The Mighty Boosh: Live in Manchester” online, opticals, vfx, titles (Warp/Universal) “No.1 Ladies Detective Agency” (BBC/HBO) assisting online conform, optical “RuBicon” Online “recap” promo (AMC) TV CAMPAIGNS “The Killer Inside Me” UK TV Campaign (Icon) “Green Zone” UK and International TV Campaign (Universal/Working Title) “Kick Ass” UK and International TV Campaign, Trailer elements (Universal/Marv -
Film Reviews
Page 78 FILM REVIEWS Kill List (Dir. Ben Wheatley) UK 2011 Optimum Releasing Note: This review contains extensive spoilers Kill List is the best British horror film since The Descent (Dir. Neil Marshall, 2005). Mind you, you wouldn’t know that from the opening halfhour or so. Although it opens with an eerie scratching noise and the sight of a cryptic rune that can’t help but evoke the unnerving stick figure from The Blair Witch Project (Dir. Daniel Myrick and Eduardo Sánchez, 1999), we’re then plunged straight into a series of compellingly naturalistic scenes of domestic discord which, as several other reviews have rightly pointed out, evoke nothing so much as the films of Mike Leigh. (The fact that much of the film’s dialogue is supplied by the cast also adds to this feeling.) But what Leigh’s work doesn’t have is the sense of claustrophobic dread that simmers in the background throughout Wheatley’s film (his second, after 2009’s Down Terrace ). Nor have any of them – to date at least – had an ending as devastating and intriguing as this. Yet the preliminary scenes in Kill List are in no sense meant to misdirect, or wrongfoot the audience: rather, they’re absolutely pivotal to the narrative as a whole, even if many of the questions it raises remain tantalisingly unresolved. Jay (Neil Maskell) and his wife Shel (MyAnna Buring) live in a spacious, wellappointed suburban home (complete with jacuzzi) and have a sweet little boy, but theirs is clearly a marriage on the rocks, and even the most seemingly innocuous exchange between them is charged with hostility and mutual misunderstanding. -
The 2019 Joint Agency Commercial Imagery Evaluation—Land Remote
2019 Joint Agency Commercial Imagery Evaluation— Land Remote Sensing Satellite Compendium Joint Agency Commercial Imagery Evaluation NASA • NGA • NOAA • USDA • USGS Circular 1455 U.S. Department of the Interior U.S. Geological Survey Cover. Image of Landsat 8 satellite over North America. Source: AGI’s System Tool Kit. Facing page. In shallow waters surrounding the Tyuleniy Archipelago in the Caspian Sea, chunks of ice were the artists. The 3-meter-deep water makes the dark green vegetation on the sea bottom visible. The lines scratched in that vegetation were caused by ice chunks, pushed upward and downward by wind and currents, scouring the sea floor. 2019 Joint Agency Commercial Imagery Evaluation—Land Remote Sensing Satellite Compendium By Jon B. Christopherson, Shankar N. Ramaseri Chandra, and Joel Q. Quanbeck Circular 1455 U.S. Department of the Interior U.S. Geological Survey U.S. Department of the Interior DAVID BERNHARDT, Secretary U.S. Geological Survey James F. Reilly II, Director U.S. Geological Survey, Reston, Virginia: 2019 For more information on the USGS—the Federal source for science about the Earth, its natural and living resources, natural hazards, and the environment—visit https://www.usgs.gov or call 1–888–ASK–USGS. For an overview of USGS information products, including maps, imagery, and publications, visit https://store.usgs.gov. Any use of trade, firm, or product names is for descriptive purposes only and does not imply endorsement by the U.S. Government. Although this information product, for the most part, is in the public domain, it also may contain copyrighted materials JACIE as noted in the text. -
Fluorescence Imaging Spectrometer (FLORIS) for ESA FLEX Mission
remote sensing Article Fluorescence Imaging Spectrometer (FLORIS) for ESA FLEX Mission Peter Coppo 1, Alessio Taiti 1, Lucia Pettinato 1, Michael Francois 2,*, Matteo Taccola 2 and Matthias Drusch 2 1 Leonardo, Via A. Einstein, 35, Campi Bisenzio, 50013 Florence, Italy; [email protected] (P.C.); [email protected] (A.T.); [email protected] (L.P.) 2 ESA ESTEC, Keplerlaan 1, P.O. Box 299, 2200 AG Noordwijk ZH, The Netherlands; [email protected] (M.T.); [email protected] (M.D.) * Correspondence: [email protected] Received: 10 May 2017; Accepted: 18 June 2017; Published: 23 June 2017 Abstract: The Fluorescence Explorer (FLEX) mission has been selected as ESA’s 8th Earth Explorer mission. The primary objectives of the mission are to provide global estimates of vegetation fluorescence, actual photosynthetic activity, and vegetation stress. FLEX will fly in tandem formation with Sentinel-3 providing ancillary data for atmospheric characterization and correction, vegetation related spectral indices, and land surface temperature. The purpose of this manuscript is to present its scientific payload, FLORIS, which is a push-broom hyperspectral imager, flying on a medium size platform. FLORIS will measure the vegetation fluorescence in the spectral range between 500 nm and 780 nm at medium spatial resolution (300 m) and over a swath of 150 km. It accommodates an imaging spectrometer with a very high spectral resolution (0.3 nm), to measure the fluorescence spectrum within two oxygen absorption bands (O2A and O2B), and a second spectrometer with lower spectral resolution to derive additional atmospheric and vegetation parameters.