Chemical Components and Aromatic Profiles of Citrus
Total Page:16
File Type:pdf, Size:1020Kb
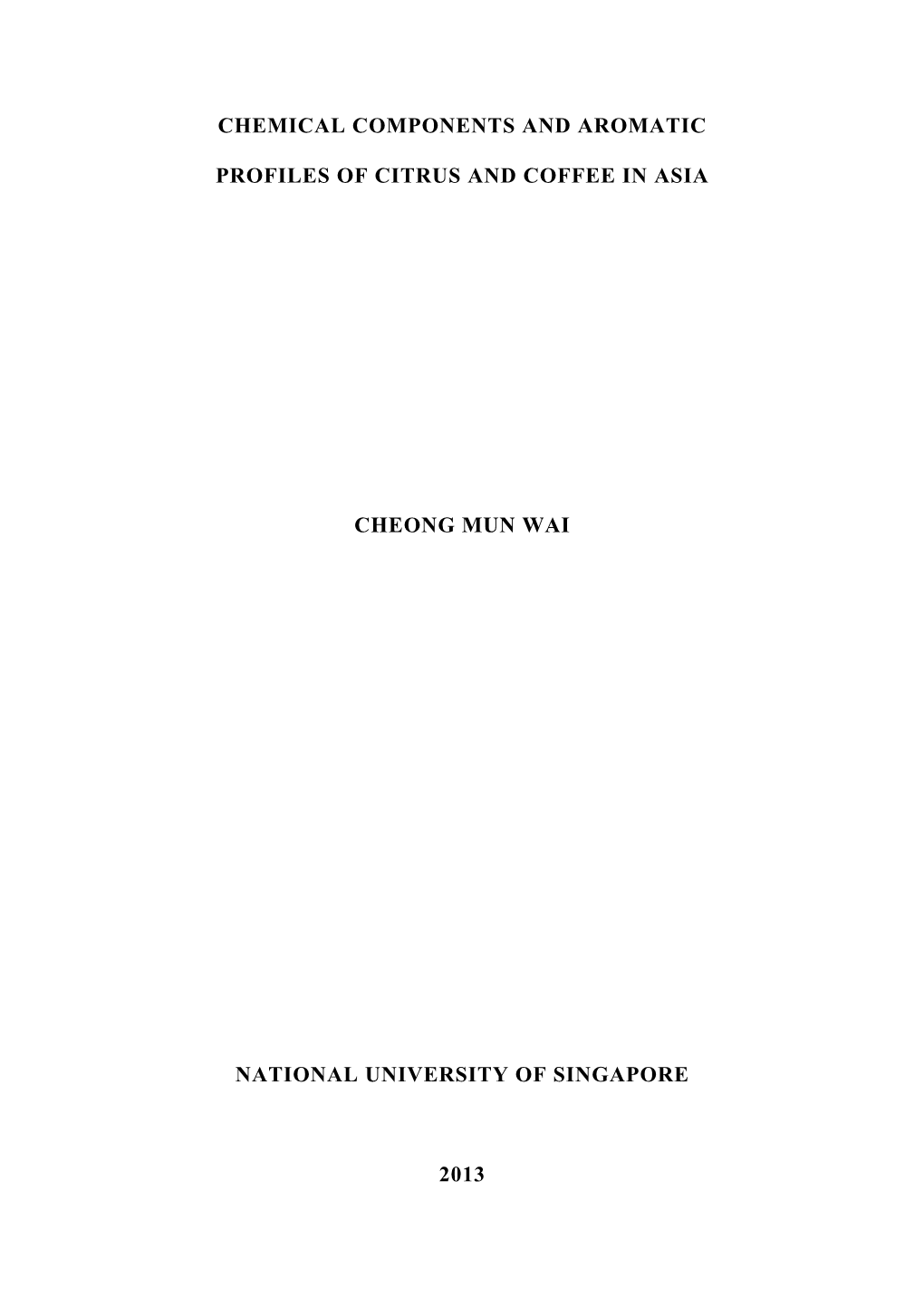
Load more
Recommended publications
-
Texas Citrus Tree Valuesjuan R
EHT-011 7/13 Texas Citrus Tree ValuesJuan R. Anciso and Luis A. Ribera* Photo courtesy of Rod Santa Ana ommercial citrus growers and homeowners To figure the total value per tree for years 2 to often need to determine the value of their 6, add the value of a tree the previous year plus mature citrus trees. The objective is to deter- the potential gross revenue the new tree is bring- mine the value of a mature grapefruit tree (Rio ing. RedC variety) and a mature orange tree (Valencia Finally, calculate the total value of mature variety) to assess the value of a tree or an orchard. trees (7 years and older) by adding the cumula- Grapefruit and orange trees usually reach full tive potential net revenue of a mature tree over 7 maturity by year 7 with an estimated yield of 23 years, plus the planting cost minus the cumulative and 18 tons per acre, respectively (Table 1.) How- potential net revenue of a new tree, years 1 to 6. ever, some production is expected from year 2 Overall, citrus tree values vary from year 1 with yearly increments until they reach maturity. through 7. At year 7, citrus trees are fully mature The price per ton used in this study is the due to their commercial production potential. Of 5-year average (2008–2012) producers received course, the longevity or lifespan of a citrus tree for either grapefruit or Valencia oranges. The cost depends on its care and whether it endures any of production is separated into planting costs or type of cold injury and rehabilitation, but the esti- establishment costs and annual production cost. -
Tropical Horticulture: Lecture 32 1
Tropical Horticulture: Lecture 32 Lecture 32 Citrus Citrus: Citrus spp., Rutaceae Citrus are subtropical, evergreen plants originating in southeast Asia and the Malay archipelago but the precise origins are obscure. There are about 1600 species in the subfamily Aurantioideae. The tribe Citreae has 13 genera, most of which are graft and cross compatible with the genus Citrus. There are some tropical species (pomelo). All Citrus combined are the most important fruit crop next to grape. 1 Tropical Horticulture: Lecture 32 The common features are a superior ovary on a raised disc, transparent (pellucid) dots on leaves, and the presence of aromatic oils in leaves and fruits. Citrus has increased in importance in the United States with the development of frozen concentrate which is much superior to canned citrus juice. Per-capita consumption in the US is extremely high. Citrus mitis (calamondin), a miniature orange, is widely grown as an ornamental house pot plant. History Citrus is first mentioned in Chinese literature in 2200 BCE. First citrus in Europe seems to have been the citron, a fruit which has religious significance in Jewish festivals. Mentioned in 310 BCE by Theophrastus. Lemons and limes and sour orange may have been mutations of the citron. The Romans grew sour orange and lemons in 50–100 CE; the first mention of sweet orange in Europe was made in 1400. Columbus brought citrus on his second voyage in 1493 and the first plantation started in Haiti. In 1565 the first citrus was brought to the US in Saint Augustine. 2 Tropical Horticulture: Lecture 32 Taxonomy Citrus classification based on morphology of mature fruit (e.g. -
Studies on the Extraction and Characterization of Pectin and Bitter
Copyright is owned by the Author of the thesis. Permission is given for a copy to be downloaded by an individual for the purpose of research and private study only. The thesis may not be reproduced elsewhere without the permission of the Author. STUDIES ON THE EXTRACTION AND CHARACTERIZATION OF' PECTIN AND BITTER PRINCIPLES FROM NEW ZEALAND GRAPEFRUIT AND PHILIPPINE CALAMANSI A thesis presented in partial fulfilment of the requirements for the d egr ee of Master of Technology in Food Technology at Massey University MYRNA ORDONA NISPEROS 1981 ii ABSTRACT A study was conducted to determine the presence of bitter components in NZ grapefruit and Philippine ca.lamansi; describe the effect of maturity on the bitter components and other chemical constituents of grapefruit; reduce the bitterness of grapefruit juice by adsorption on polyvinylpyrrolidone; and to extract and characterize pectin from grapefruit peel. Naringin (995 ppm), narirutin (187 ppm), and limonoids (7.9 ppm) were detected in NZ grapefruit juice concentrate (27° Brix). Naringin was not detected in the calamansi juice, and limonin was detected at the level of 10.5 ppm in juice containing 5% crushed seeds. Maturation of the grapefruit caused an increase in pH from J.00 to J.50, an increase in total soluble solids from 10.8 to 14.4 with a decline to 13.5° Brix later in the season, a steady fall in acidity from 2.50 to 1.31 g citric acid/100 mL, and a continuous rise in the Brix/acid ratio from 4.2 to 10.J. Juice yield fluctuated throughout the season. -
Citrus Industry Biosecurity Plan 2015
Industry Biosecurity Plan for the Citrus Industry Version 3.0 July 2015 PLANT HEALTH AUSTRALIA | Citrus Industry Biosecurity Plan 2015 Location: Level 1 1 Phipps Close DEAKIN ACT 2600 Phone: +61 2 6215 7700 Fax: +61 2 6260 4321 E-mail: [email protected] Visit our web site: www.planthealthaustralia.com.au An electronic copy of this plan is available through the email address listed above. © Plant Health Australia Limited 2004 Copyright in this publication is owned by Plant Health Australia Limited, except when content has been provided by other contributors, in which case copyright may be owned by another person. With the exception of any material protected by a trade mark, this publication is licensed under a Creative Commons Attribution-No Derivs 3.0 Australia licence. Any use of this publication, other than as authorised under this licence or copyright law, is prohibited. http://creativecommons.org/licenses/by-nd/3.0/ - This details the relevant licence conditions, including the full legal code. This licence allows for redistribution, commercial and non-commercial, as long as it is passed along unchanged and in whole, with credit to Plant Health Australia (as below). In referencing this document, the preferred citation is: Plant Health Australia Ltd (2004) Industry Biosecurity Plan for the Citrus Industry (Version 3.0 – July 2015). Plant Health Australia, Canberra, ACT. Disclaimer: The material contained in this publication is produced for general information only. It is not intended as professional advice on any particular matter. No person should act or fail to act on the basis of any material contained in this publication without first obtaining specific and independent professional advice. -
Citrus Fruits Information Compiled by Sunkist Growers
Commodity Fact Sheet Citrus Fruits Information compiled by Sunkist Growers How Produced – Citrus trees are propagated asexually mandarin orange and a lemon, are less acidic than traditional through a procedure known as grafting which fuses two lemons. different varieties of plants. In the case of citrus trees, one variety, the rootstock, is selected for Desert grapefruit are harvested October its hardiness and the other variety, the through March while summer grapefruit scion, is selected for its high-quality are available May through September. fruits. The rootstock, grown from a Specialty citrus include Melo Golds seed, is typically a two- to three-year- and Oro Blancos, grapefruit varieties old seedling while the scion is a bud that are popular with those preferring a from a mature tree. Through grafting, sweeter taste. Pummelos, or “Chinese” the scion fuses to the rootstock and grapefruit, considered a delicacy among becomes a new tree. In approximately many Asian cultures, are the largest of five years, the tree produces the same all citrus fruits. variety of fruit that was budded onto Almost a dozen different mandarin the rootstock. The successfully grafted and tangerine varieties, such as trees are sold to citrus growers through Clementines, Gold Nuggets, and wholesale nurseries and are certified Pixies, are available November through disease-free. There are approximately CITRUS VARIETIES May. Most are easy to peel and have a 270,000 bearing acres of citrus trees in lively flavor. California. Commodity Value – While Florida is the number one History – Oranges and lemons can be traced back to the producer of citrus fruits, the majority of their crop is made ancient Middle East. -
Holdings of the University of California Citrus Variety Collection 41
Holdings of the University of California Citrus Variety Collection Category Other identifiers CRC VI PI numbera Accession name or descriptionb numberc numberd Sourcee Datef 1. Citron and hybrid 0138-A Indian citron (ops) 539413 India 1912 0138-B Indian citron (ops) 539414 India 1912 0294 Ponderosa “lemon” (probable Citron ´ lemon hybrid) 409 539491 Fawcett’s #127, Florida collection 1914 0648 Orange-citron-hybrid 539238 Mr. Flippen, between Fullerton and Placentia CA 1915 0661 Indian sour citron (ops) (Zamburi) 31981 USDA, Chico Garden 1915 1795 Corsican citron 539415 W.T. Swingle, USDA 1924 2456 Citron or citron hybrid 539416 From CPB 1930 (Came in as Djerok which is Dutch word for “citrus” 2847 Yemen citron 105957 Bureau of Plant Introduction 3055 Bengal citron (ops) (citron hybrid?) 539417 Ed Pollock, NSW, Australia 1954 3174 Unnamed citron 230626 H. Chapot, Rabat, Morocco 1955 3190 Dabbe (ops) 539418 H. Chapot, Rabat, Morocco 1959 3241 Citrus megaloxycarpa (ops) (Bor-tenga) (hybrid) 539446 Fruit Research Station, Burnihat Assam, India 1957 3487 Kulu “lemon” (ops) 539207 A.G. Norman, Botanical Garden, Ann Arbor MI 1963 3518 Citron of Commerce (ops) 539419 John Carpenter, USDCS, Indio CA 1966 3519 Citron of Commerce (ops) 539420 John Carpenter, USDCS, Indio CA 1966 3520 Corsican citron (ops) 539421 John Carpenter, USDCS, Indio CA 1966 3521 Corsican citron (ops) 539422 John Carpenter, USDCS, Indio CA 1966 3522 Diamante citron (ops) 539423 John Carpenter, USDCS, Indio CA 1966 3523 Diamante citron (ops) 539424 John Carpenter, USDCS, Indio -
Calamansi Puree Spec Sheet
PacRim puree CALAMANSI NATURAL FRUIT PUREE Located in the prized citrus region of Can Tho, Vietnam is the co-operative of growers that loosely goes by name of Duong-Vu, representing the last names of Nutrition Facts the two prominent families in the area. Calamansi, technically a citrofortunella, 32 servings per container Serving size 1oz (28g) meaning it is a hybrid of the citrus and kumquat families and is highly acidic. Prized for its bright floral aromas and a very tart profile. In Can Tho, calamansi Amount per serving is available year round and is harvested when the fruit is still slightly immature. Calories 20 % Daily Value* Total Fat 0g 0% Saturated Fat 0g 0% Trans Fat 0g Cholesterol 0mg 0% Sodium 0mg 0% Origin: Can Tho, Vietnam Brix: 10 Total Carbohydrate 2g 1% Dietary Fiber 1g 4% pH: 2.5 FLAVOR PROFILE Total Sugars 1g Ingredients: Calamansi Fruit, Water, Cane Sugar Includes 1g Added Sugars 2% Nose: Bright, floral aroma of unripened Protein 0g 0% citrus peel and wheatgrass Handling: Keep Frozen Flavor: Tart, high acidity, sour mandarin Packaging: 32oz x 6 – White plastic cylinder Vitamin D 0mcg 0% orange-like with notes of key lime with label and screw-on cap with safety seal Calcium 13mg 0% Iron 0.18mg 0% and pith Jar Dims: 3 1/2 x 3 1/2 x 6 1/2 ” ” ” Potassium 0mg 0% SPECIFICATIONS Case Dims: 11 1/8” x 7 7/16” x 6 3/4” – 12lbs Vitamin A 0% Vitamin C 20% Process: Proprietary minimal whole fruit * The % Daily Value (DV) tells you how much a nutrient in process, presented in its natural state, a serving of food contributes to a daily diet. -
Improvement of Subtropical Fruit Crops: Citrus
IMPROVEMENT OF SUBTROPICAL FRUIT CROPS: CITRUS HAMILTON P. ÏRAUB, Senior Iloriiciilturist T. RALPH ROBCNSON, Senior Physiolo- gist Division of Frnil and Vegetable Crops and Diseases, Bureau of Plant Tndusiry MORE than half of the 13 fruit crops known to have been cultivated longer than 4,000 years,according to the researches of DeCandolle (7)\ are tropical and subtropical fruits—mango, oliv^e, fig, date, banana, jujube, and pomegranate. The citrus fruits as a group, the lychee, and the persimmon have been cultivated for thousands of years in the Orient; the avocado and papaya were important food crops in the American Tropics and subtropics long before the discovery of the New World. Other types, such as the pineapple, granadilla, cherimoya, jaboticaba, etc., are of more recent introduction, and some of these have not received the attention of the plant breeder to any appreciable extent. Through the centuries preceding recorded history and up to recent times, progress in the improvement of most subtropical fruits was accomplished by the trial-error method, which is crude and usually expensive if measured by modern standards. With the general accept- ance of the Mendelian principles of heredity—unit characters, domi- nance, and segregation—early in the twentieth century a starting point was provided for the development of a truly modern science of genetics. In this article it is the purpose to consider how subtropical citrus fruit crops have been improved, are now being improved, or are likel3^ to be improved by scientific breeding. Each of the more important crops will be considered more or less in detail. -
Common and Botanical Names of Some Species and Hybrids of Citrus and Citrus Relatives Mentioned in the Proceedings*
Common and Botanical Names of Some Species and Hybrids of Citrus and Citrus Relatives Mentioned in the Proceedings* Aeglopsis chevalieri Swing. Kumquat (Marumi, Luowen) Alemow I? japonica (Thumb.) Swing. Citrus macrophylla Webster Kumquat (Nagami, Luofu) Atalantia sp. D.C. F. margarita (Lour.) Swing. Balsamocitrus dawei Stapf Lemon Bergamot Eureka, Eureka cascade, Femminello Sir- C. bergamia Risso and Poit. acusano, Femminello Fior d'arancio, Calamondin Frost Lisbon, Hong-li-meng, Lapithos, Calamansi Ross Eureka, Tu-li-meng C. madurensis Lour. = C. mitis Blanco C. limon (L.) Burn.f. Chironja Lime C. paradisi hybrid Key, Mexican, West Indian Citrange C. aurantifolia (Christm.) Swing. Carrizo, Rusk, Troyer, Yuma Lime C. sinensis x Poncirus trifoliata Bearss, Persian, Tahiti Citron C. latifolia Tan. Etrog Arizona 861-5-1 Limetta C. medica L. Sweet Citrumelo C. limetta Risso Swingle Mandarins and hybrids C. paradisi x P. trifoliata Angeru, Baillianzi, Balye 2, Beauty, Ben- C. bigaradia = C. aurantium L. dizhao, Canh, Cha-zhi-gan, Coorg, C. deliciosa Ten. Cravo, Dancy, Duong, Ellendale, Fiju, C. erythrosa Hort. ex. Tan. Harry, Hong Nhieu, Hongju, Kara, San-hu-hong-ju, Zhuju King, Kinnow, Ladu, Loose Jacket, C. excelsa Webster Mangju, Mexerica do Rio, Mexerica C. halimii Stone Tardia A, Miyakawa, Murcott, Nagpur, C. succosa Hort. ex. Tan. Nan-feng-mi-ju, New Ben No.1, Nova, C. sunki Hort. ex. Tan. Oneco, Parson's special, Ponkan, Ruju, Suanyu Shaohe Ponkan, Shehui kan, Som-keo- Clementine wan, Som-pan, Szinkon, Tankan, Algerian tangerine, Arrufatina, Com- Tianma Ponkan, Thieu, Thorney Zaoju, mune, Fina, Monreal, Nules, Oroval Wenmi, Willowleaf, Yanhou Ponkan, C. clementina Hort. -
New Variety Highlights
18/12/2017 New variety highlights DPI Roadshow, Loxton 2017 G. Sanderson, D. Monks, T. Witte and A. Creek NSW DPI & K. Lacey Dept. Primary Industries and Regional Development, WA (19th Oct. 2017, Loxton) Top work October 2017 First field fruit in 2018 . Gusocora Valencia . Midknight Valencia 115-1717 . Greenwood navel orange . Ruby Valencia . 1474 mandarin . Mclean Valencia . 1614 mandarin . Lavalle Valencia . 1627 mandarin . Benny Valencia . 1848 mandarin . Rayno navel orange . AC41114LS Afourer mandarin . Glen Ora navel orange . HC Afourer mandarin . Witkrans navel orange . H2 seedless mandarin . 91-03-04 mandarin . MJR11 mandarin (early Nova) . USDA 88-2 mandarin . MJR 12 mandarin (early Nova) . Star Ruby grapefruit (early) . Star Ruby grapefruit (early) . Star Ruby grapefruit (late) . Star Ruby grapefruit (late) . Jackson grapefruit . Italian lemon 1 18/12/2017 Local selections v’s imported Joe’s Early Turkey Valencia Early season orange maturity comparison Dareton 12th September 2017 Variety Juice % ºBrix Acid% B:A BrimA Joe’s Early 46 12.7 0.77 16.4 158 Turkey Valencia 48 11.5 1.13 10.2 115 Midknight Valencia 52 12.7 1.10 11.5 137 2 18/12/2017 Dolci navel July 2017 Documentary series Legends of Fruit (Navel orange) 3 18/12/2017 RHM (Royal Honey Murcott) RHM mandarin Estimated maturity period Region Jan Feb Mar April May June July Aug Sep Oct Nov Dec Sunraysia Date Variety Juice% ºBrix Acid% B:A BrimA 26/6/2017 RHM 56 11.7 0.54 21.7 157 4 18/12/2017 Tarocco Ippolito blood orange Tarocco Ippolito budwood Auscitrus - Buds sold 25000 20000 15000 Buds no. -
Citrus Varieties in Egypt: an Impression
International Research Journal of Applied Sciences Short Communication pISSN: 2663-5577, eISSN: 2663-5585 Citrus Varieties in Egypt: An Impression Waleed Fouad Abobatta Department of Citrus, Horticulture Research Institute, Agriculture Research Center, Egypt ARTICLE INFORMATION ABSTRACT Received: October 30, 2018 Citrus industry is very important for Egyptian economy, citrus fruit is the leading exportable agricultural product of Egypt and is an important source of national income. Citrus cultivation Accepted: December 03, 2018 area represents about 29% of the total fruit area, there are different citrus varieties cultivated in Egypt. This work aims to provide a short description of main citrus varieties cultivated in Published: January 31, 2019 Egypt through providing information about fruit size, maturity periods, seediness and productivity average and main cultivated areas for each variety. However Washington Navel Corresponding Author: and Valencia orange are the main varieties followed by Mandarins group varieties, lemon, Waleed Fouad Abobatta, Balady orange, while other varieties like Grapefruit, Sour orange and Kumquat are cultivated Department of Citrus, in small areas. Horticulture Research Institute, Agriculture Research Center, Egypt Key words: Citrus industry, navel orange, valencia orange, mandarins group, citrus varieties INTRODUCTION Citrus is a genus from Rutaceae family, subfamily Aurantoideae1 and there are several species in this genus; but there are major species such as sweet orange (Citrus sinensis (L.) Osbeck), mandarins group, grapefruits (Citrus paradisi ), lime (Citrus aurantifolia) and sour orange (Citrus aurantium L.)2. Citrus is a diploid genus origin in tropical, subtropical, but now it is produced mainly in arid and semiarid regions. Citrus species are among the most widely grown fruit crops in the world and have a huge market all over the world3. -
CITRUS BUDWOOD Annual Report 2017-2018
CITRUS BUDWOOD Annual Report 2017-2018 Citrus Nurseries affected by Hurricane Irma, September 2017 Florida Department of Agriculture and Consumer Services Our Vision The Bureau of Citrus Budwood Registration will be diligent in providing high yielding, pathogen tested, quality budlines that will positively impact the productivity and prosperity of our citrus industry. Our Mission The Bureau of Citrus Budwood Registration administers a program to assist growers and nurserymen in producing citrus nursery trees that are believed to be horticulturally true to varietal type, productive, and free from certain recognizable bud-transmissible diseases detrimental to fruit production and tree longevity. Annual Report 2018 July 1, 2017 – June 30, 2018 Bureau of Citrus Budwood Registration Ben Rosson, Chief This is the 64th year of the Citrus Budwood Registration Program which began in Florida in 1953. Citrus budwood registration and certification programs are vital to having a healthy commercial citrus industry. Clean stock emerging from certification programs is the best way to avoid costly disease catastrophes in young plantings and their spread to older groves. Certification programs also restrict or prevent pathogens from quickly spreading within growing areas. Regulatory endeavors have better prospects of containing or eradicating new disease outbreaks if certification programs are in place to control germplasm movement. Budwood registration has the added benefit in allowing true-to-type budlines to be propagated. The selection of high quality cultivars for clonal propagation gives growers uniform plantings of high quality trees. The original mother stock selected for inclusion in the Florida budwood program is horticulturally evaluated for superior performance, either by researchers, growers or bureau staff.