High Energy Colliders and the Rise of the Standard
Total Page:16
File Type:pdf, Size:1020Kb
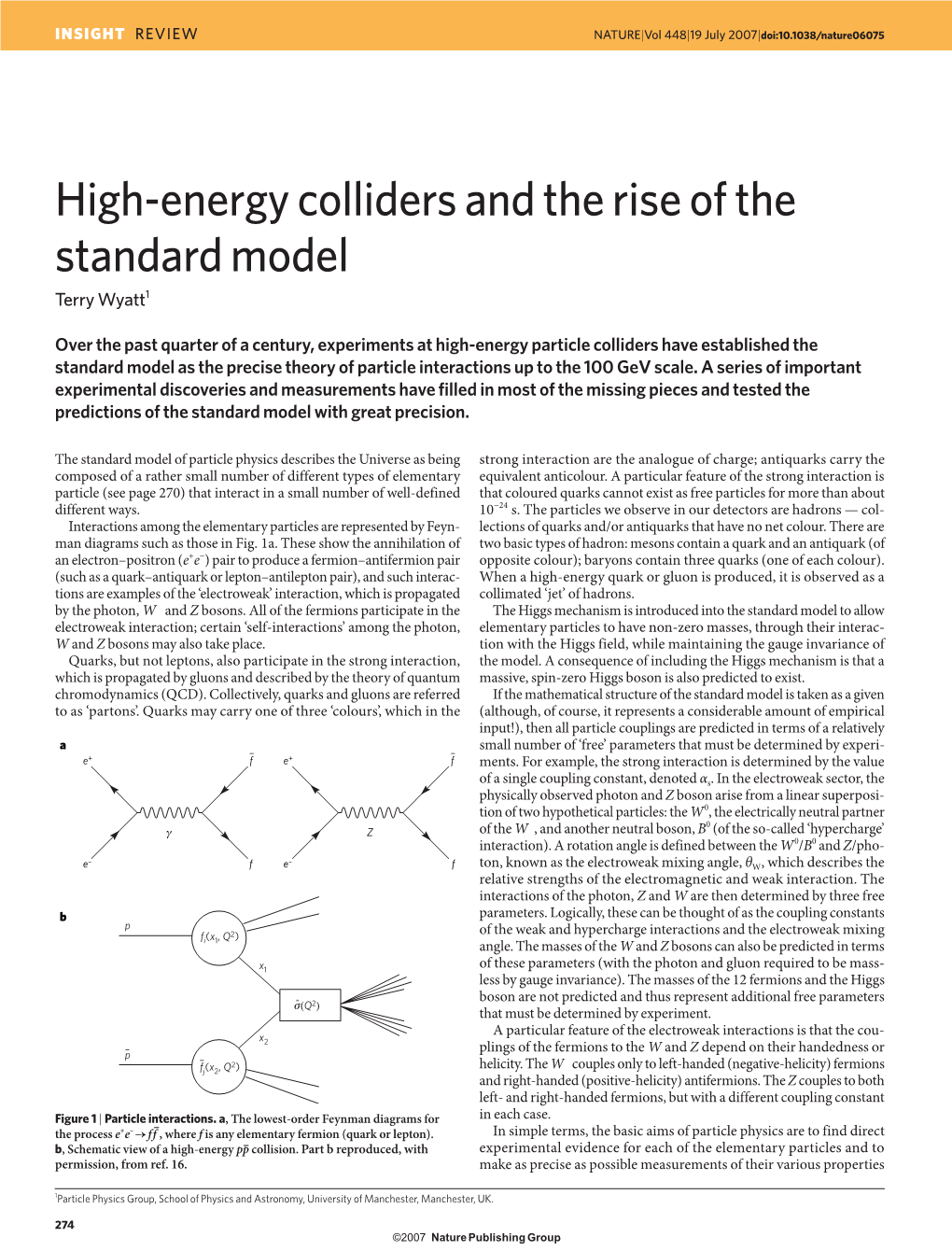
Load more
Recommended publications
-
Prof. Terry Wyatt FRS Employment History Education Fellowships And
CURRICULUM VITAE — Prof. Terry Wyatt FRS Born: 29th June 1957, Watford, England. Nationality: British. Family Status: Married, with two sons. Employment History Tenured Physics Faculty: School of Physics and Astronomy, University of Manchester, UK. – Professor (2004–present) [DØ and ATLAS experiments] – Reader (1999–2004) [OPAL and DØ experiments] – Lecturer (1996–1999) [OPAL experiment] – Senior visiting appointments, on leave from usual academic duties at University of Manchester: Guest Scientist, Fermilab: 15 month period as Paid Guest Scientist at Fermilab (2002–2003) PPARC Senior Research Fellowship: 3-year period (2003-2006) STFC support for DØ spokesperson: 100% salary buy-out for 18 month period (2006–2007). As spokesperson of DØ I also received financial support from Fermilab. – I currently serve as Chair of the Accelerator, Nuclear and Particle Physics Division of the School of Physics and Astronomy, with overall responsibility for both experimental and theoretical aspects of these fields. PPARC Advanced Research Fellow: Based at CERN with University of Manchester, UK. (1989–1996). [OPAL experiment]. PPARC Research Associate: QMC, University of London, UK. (1986–1989). [OPAL experiment] CERN Fellow: CERN, Geneva, Switzerland. (1984–1986). [UA1 experiment] Education Research Student: University of Oxford, UK. (1979–1983). [TASSO experiment] Degree: D.Phil. Undergraduate Student in Physics: Imperial College, University of London, UK. (1976–1979). Degree: B.Sc. (Ist class hons. Awarded governors’ prize for top first in physics, 1979). Associateship of the Royal College of Science (A.R.C.S). Fellowships and Prizes • Elected as Fellow of the Royal Society, FRS (2013). Excerpt from citation: “Distinguished for a number of original and important contributions to the experimental verification of the Standard Model”. -
Sandbox Studio 9 Symme
it on symmetry | volume 03 issue 10 december 06 Photos: Sandbox Studio 9 Physicist Michael Weber (right, Fermilab) and his colleagues (from left) Michael Kirby (Radboud University Nijmegen, Netherlands), Chris Neu (University of Pennsylvania), Sabine Lammers (Columbia University), Ben Kilminster (Ohio State University) as well as other experimenters at CDF and DZero are hitting upon numerous discoveries. Photo: Sandbox Studio As work continues to complete the Large Hadron Collider in Europe and plans develop around the world for an International Linear Collider, one acceler- ator at the energy frontier is open for business right now. At Fermilab in Batavia, Illinois, the Tevatron collider is making discoveries. Michael Weber is working late this Tuesday night in mid- November, crunching data from his desk in a cubicle in Fermilab’s DZero building. He’s analyzing data collected by the DZero collider experiment. He is determined to wring every drop of information from the experiment’s vast and growing hoard of data. Just across the Tevatron ring, the scene is similar for Laura Sartori, working on the CDF experi- ment. Michael and Laura are looking for discoveries that could change particle physics forever. And the clock is ticking. Michael and Laura are not alone—far from it. Almost 1400 sci- entists, members of the CDF and DZero collaborations, are pouring on the effort to make the most of the US accelerator’s final run before CERN’s Large Hadron Collider takes over the energy frontier later in the decade. Long after normal working hours, the lights burn in the cubicles of the CDF and DZero offices as experimenters search for the tell-tale tracks that might lead them toward a sighting of supersymmetry, extra dimensions, dark matter, exotic particles, and a host of other phenomena that no one on the planet has ever seen before. -
Research Resumé — Prof. Terry Wyatt
Research Resum´e— Prof. Terry Wyatt FRS The Standard Model (SM) of particle physics describes the interactions of the gauge bosons (γ, Z, W±, gluons) with one another and with the fundamental fermions (the quarks and leptons). Over the past thirty years I have made a number of influential measurements at electron-positron and hadron- hadron colliders that have helped establish the SM as the precise theory of the elementary particles. My work has enabled significant improvements in the experimental precision with which: (a) the SM has been tested and (b) models of the possible new physics beyond the SM (BSM) have been constrained. In order to make these measurements I have developed a number of innovative techniques that have continued to find wide application within the field. In recognition of my contributions to hadron collider physics I was awarded the 2011 Chadwick medal and prize by the Institute of Physics. I was elected as a Fellow of the Royal Society (FRS) in 2013. My D.Phil. research was performed with the TASSO experiment at what was then the world’s highest energy e+e− collider, PETRA at DESY. This was before the discovery of the W and Z bosons and I chose to work on testing a very important prediction of the SM, namely that fermion-antifermion pairs should be produced with a forward-backward charge asymmetry in e+e− annihilation (arising from Z/γ interference). I made the first observation of this asymmetry in the bb final state. In order to make this measurement I developed the first successful algorithm to use kinematic information to distinguish b quark jets from the jets produced by other, lighter, quarks. -
ELECTROWEAK MEASUREMENTS from RUN II at the TEVATRON TR WYATT Department of Physics and Astronomy, University of Manchester
ELECTROWEAK MEASUREMENTS FROM RUN II AT THE TEVATRON T. R. WYATT Department of Physics and Astronomy, University of Manchester, Manchester M13 9PL, UK Email: [email protected] The CDF and DÂ detectors were fully commissioned for physics running in Run II at the Tevatron pp¹ collider in early 2002. Since then both experiments have collected data samples corresponding to an integrated luminosity of around L = 200 pb¡1 at a pp¹ centre-of-mass energy of ps = 1.96 TeV. Datasets corresponding L = 120 pb¡1 have beenR analyzed for physics so far. Recent electroweak measurements from Run II are reviewed.R Cross section times branching ratio measurements (σ Br) are presented for the intermediate vector bosons (IVB's) in their leptonic ¢ decay modes: W `º and Z `+`¡. For the ¯rst time, a combination of the σ Br results from the CDF and DÂ ! ! ¢ experiments is made; this includes using a consistent choice of the total inelastic pp cross section for the luminosity determinations of the two experiments. Quantities derived from these σ Br values are also updated. These include: ¢ R the ratio of the σ Br values for W and Z; Br(W `º), the leptonic branching ratio of the W ; and ¡W, the total ` ¢ ! decay width of the W . Other measurements using events containing W and Z leptonic decays are presented, including studies that probe the QCD phenomenology of W /Z production and searches for events containing two intermediate vector bosons. 1. Experimental Measurements of σ ¢ Br for Z ! `+`¡ and W ! `º 1.1. Introduction Figure 1 shows the mechanism for IVB production in pp¹ collisions. -
Belief and Observation: the Top Quark and Other Tales of “Discovery”
Belief and Observation: The Top Quark and Other Tales of “Discovery” T. Ferbel Universities of Rochester and Maryland “Seek, and Ye Shall Find” (Matthew 7:7) (If you search long enough, you’re bound to find something!) 1 Plan for the evening: • Story of the discovery of the top quark (1995) • Story of the stillbirth of the b* meson (1964) • Tragedy of the “splitting” of the A2 meson (≈1970) • A tale from “Pathological Science” (Langmuir ≈1930) • A few words on medical research!!! • Skip many other quirks: cold fusion, gravity waves, βν>1, N-rays, leptoquarks at DESY, “OopsLeon,“ etc, etc, from work of top scientists over past 100 years! • A word on human frailty (but hope) --------------------------- References: I. Langmuir, A. Wróblewski, S. Stone, others on web. 2 History of the top quark 1973: Makoto Kobayashi and Toshihide Maskawa predict the existence of a third generation of quarks in their attempt to accommodate the observed violation of CP invariance in K0 decays. (Cabibbo/GIM mixing combine in CKM!) 1974: The November revolution with discovery of the J/ψ and the fourth (GIM) “charm” quark at both BNL and SLAC by Sam Ting et al and Burt Richter et al, respectively, and, shortly thereafter, the τ lepton by Martin Perl et al (also at SLAC), with the τ providing major support for a third generation of fermions. 1975: Haim Harari, a great wizard (Israeli theorist) of the era, names the quarks of the third generation "top" and "bottom" to match the "up" and "down" quarks of the first generation, reflecting their "spin up" and "spin down" membership in a new weak-isospin doublet that also restores the numerical quark/lepton symmetry of the next (current) version of the standard model. -
Arxiv:1312.4884V1 [Physics.Soc-Ph] 17 Dec 2013 Snowmass 2013 Electronic Proceedings Community Summer Study, Minneapolis, MN July 29 – August 6, 20123 Contents
SLAC{PUB{15859 December, 2013 Benefits to the U.S. from Physicists Working at Accelerators Overseas Jacob Anderson, Raymond Brock, Yuri Gershtein, Nicholas Hadley, Michael Harrison, Meenakshi Narain, Jason Nielsen, Fred Olness, Bjoern Penning, Michael Peskin, Eric Prebys, Marc Ross, Salvatore Rappoccio, Abraham Seiden, Ryszard Stroynowski ABSTRACT We illustrate benefits to the U.S. economy and technological infrastructure of U.S. participation in accelerators overseas. We discuss contributions to exper- imental hardware and analysis and to accelerator technology and components, and benefits stemming from the involvement of U.S. students and postdoctoral fellows in global scientific collaborations. CONTRIBUTED TO arXiv:1312.4884v1 [physics.soc-ph] 17 Dec 2013 Snowmass 2013 Electronic Proceedings Community Summer Study, Minneapolis, MN July 29 { August 6, 20123 Contents 1 Introduction 2 2 U.S. Leadership in Fundamental Science 3 2.1 Today's Big Science Scale . 3 2.2 Sharing of effort in large science projects . 5 2.3 Sharing international responsibility for large projects . 7 3 Particle Physics Innovation-Transfer 7 3.1 Contributions to Detectors . 8 3.1.1 CMS endcap . 9 3.1.2 ATLAS pixel tracker . 9 3.2 Contributions to Accelerators . 11 3.2.1 Accelerator collaboration in overseas projects . 11 3.2.2 The LHC luminosity upgrade . 13 3.2.3 The ILC R&D program . 14 3.2.4 Accelerator innovation: conclusions . 15 4 Particle Physics Imagination-Transfer 16 4.1 Contributions to Experimental Analysis . 16 4.1.1 An analysis contributor { 1 . 16 4.1.2 An analysis contributor { 2 . 17 4.1.3 An analysis leader . -
Evaluation of the Benefits That the UK Has Derived from CERN
Evaluation of the benefits that the UK has derived from CERN Evidence Document August 2020 Evaluation of the benefits that the UK has derived from CERN Evidence Document technopolis |group| July 2020 Neil Brown Paul Simmonds Cristina Rosemberg Maike Rentel Charlotte Glass Antonella De Santo Table of Contents 1 Introduction .............................................................................................................................................. 1 2 Study scope and objectives ....................................................................................................................... 2 3 Approach and methods ............................................................................................................................ 2 4 CERN, its facilities and activities ............................................................................................................. 4 4.1 History and governance ........................................................................................................................ 4 4.2 Facilities and capabilities ...................................................................................................................... 5 4.3 Technology development ...................................................................................................................... 9 4.4 Contracts and procurement .................................................................................................................11 4.5 Knowledge transfer ............................................................................................................................. -
Overview of Terry's 2019
Overview of Terry’s 2019 Terry Wyatt. Manchester Christmas Meeting, 2019. Current PhD students • Sam Dysch: search for τ-lepton universality violation in W decays – Target precision ~1.5% for Run 2 data set (c.f. LEP-combined: 6.5%) • Diego Barón: τ-lepton identification at high pT • Lewis Higgins: Football (co-supervised with Tobias Galla) – EPSRC CASE – co-funded with Man.City) • Plus MPhys students (fysics and phootball) • Plus 10 fb-1 of 13 TeV ATLAS data in the 3rd year UG teaching lab. – First evidence for Higgs to 4-leptons in the 3rd year lab.! 2 Football • Data available: every game in 2016, 2017, 2018 and current premier league seasons • Technical work on datasets (in close collaboration with City expert) • Store in a consistent fashion data from different sources: position of players and ball, ball possession, game events • Now in a position to repeat/combine/extend studies previously performed as part of MPhys/summer projects Figure 7.14: Premier League 2017 - Optimised tiles on a per match basis. Velocity distorted, nearest player. Spatial Control: Voronoi regions boosted by player Correlation between “expected velocity and including consideration goals” and ”spatial control” of contested space 3 Figure 7.11: Diagram of the pixels created by the distorted Voronoi definition combined with the contested pixels (nearest home, nearest away) model. The distortedFigure Voronoi 7.15: model Premiercan be used League to modify 2018the standard - Optimised definition of tiles the Voronoi on a (nearest per match basis. Velocity distorted, nearest player. player by time, rather than distance) - figure 7.10 is an example of this. -
5Th International Masterclasses „Hands on Particle Physics“
EPPOG International Masterclasses “Hands on Particle Physics“ www.physicsmasterclasses.org Plenary ECFA meeting, CERN, 26.11.2009 Michael Kobel, TU Dresden The masterclass idea Basic idea from UK Students (16-19 year-olds) spend 1 day at research institute Listen to scientists‘ introduction to particle physics Work like real scientists: measurements with particle physics data Why Masterclasses? Make modern particle physics data available to students Let students explore fundamental forces and building blocks of nature Demonstrate the scientific research process Stimulate interest in science The EPPOG international masterclass idea Create an international collaboration of students Join institutes worldwide with 300-400 students per day Since 2005: all w/in 3 weeks each year Over 80 institutes from 23 countries taking part Over 6000 students participating 5-6 masterclasses join in video conference at the end of the day Discuss results and differences Combine results (better accuracy) Quiz and prizes Questions to scientists schedule, data, background material, description of all participating institutes: www.physicsmasterclasses.org Participation EPPOG international masterclasses 25 120 20 100 80 15 60 10 40 5 20 0 0 2005 2006 2007 2008 2009 2005 2006 2007 2008 2009 Countries Institutes US 6000 (Quarknet) 5000 4000 3000 2000 1000 Brazil 0 2005 2006 2007 2008 2009 South Africa Participants Examples: Italy, Germany Hamburg Berlin Göttingen WuppertalDortmund Dresden Aachen Siegen Bonn Würzburg Mainz Erlangen Heidelberg Tübingen München Freiburg 2009: 14 institutes 2010: +2 institutes Agenda of the day Lectures Standard model, Accelerators, Detectors institute´s activities, cosmology etc. Lunch with physics students and tutors Measurements Video conference Lectures Measurements Collecting results Local analysis Final video conference: combination of results Quiz with the CERN moderators Prizes funded by CERN Masterclass CD-rom 10 web-based systems with material in 17 languages online at http://www.physicsmasterclasses.org physics offline on masterclass CDrom (ca. -
Membership of Sectional Committees 2015
Membership of Sectional Committees 2015 The main responsibility of the Sectional Committees is to select a short list of candidates for consideration by Council for election to the Fellowship. The Committees meet twice a year, in January and March. SECTIONAL COMMITTEE 1 [1963] SECTIONAL COMMITTEE 3 [1963] Mathematics Chemistry Chair: Professor Keith Ball Chair: Professor Anthony Stace Members: Members: Professor Philip Candelas Professor Varinder Aggarwal Professor Ben Green Professor Harry Anderson Professor John Hinch Professor Steven Armes Professor Christopher Hull Professor Paul Attfield Professor Richard Kerswell Professor Shankar Balasubramanian Professor Chandrashekhar Khare Professor Philip Bartlett Professor Steffen Lauritzen Professor Geoffrey Cloke Professor David MacKay Professor Peter Edwards Professor Robert MacKay Professor Malcolm Levitt Professor James McKernan Professor John Maier Professor Michael Paterson Professor Stephen Mann Professor Mary Rees Professor David Manolopoulos Professor John Toland Professor Paul O’Brien Professor Srinivasa Varadhan Professor David Parker Professor Alex Wilkie Professor Stephen Withers SECTIONAL COMMITTEE 2 [1963] SECTIONAL COMMITTEE 4 [1990] Astronomy and physics Engineering Chair: Professor Simon White Chair: Professor Hywel Thomas Members: Members: Professor Girish Agarwal Professor Ross Anderson Professor Michael Coey Professor Alan Bundy Professor Jack Connor Professor Michael Burdekin Professor Laurence Eaves Professor Russell Cowburn Professor Nigel Glover Professor John Crowcroft -
Springer Theses
Springer Theses Recognizing Outstanding Ph.D. Research For further volumes: http://www.springer.com/series/8790 Aims and Scope The series ‘‘Springer Theses’’ brings together a selection of the very best Ph.D. theses from around the world and across the physical sciences. Nominated and endorsed by two recognized specialists, each published volume has been selected for its scientific excellence and the high impact of its contents for the pertinent field of research. For greater accessibility to non-specialists, the published versions include an extended introduction, as well as a foreword by the student’s supervisor explaining the special relevance of the work for the field. As a whole, the series will provide a valuable resource both for newcomers to the research fields described, and for other scientists seeking detailed background information on special questions. Finally, it provides an accredited documentation of the valuable contributions made by today’s younger generation of scientists. Theses are accepted into the series by invited nomination only and must fulfill all of the following criteria • They must be written in good English. • The topic should fall within the confines of Chemistry, Physics, Earth Sciences, Engineering and related interdisciplinary fields such as Materials, Nanoscience, Chemical Engineering, Complex Systems and Biophysics. • The work reported in the thesis must represent a significant scientific advance. • If the thesis includes previously published material, permission to reproduce this must be gained from the respective copyright holder. • They must have been examined and passed during the 12 months prior to nomination. • Each thesis should include a foreword by the supervisor outlining the signifi- cance of its content. -
DØ Status and Prospects
DØ Status and Prospects Terry Wyatt, University of Manchester Dmitri Denisov, Fermilab June 8 2007, P5 Meeting at Fermilab Executive Summary • DØ detector – Run IIb upgrade is a success – Smoothly collecting physics data – 2.5fb-1 recorded – No technical issues through 2009 expected • Triggering and data reconstruction – Full high Pt trigger menu up to highest luminosities – Reconstruction is keeping pace with data collection • The DØØp ph ysics p ro gram – 2006 is the best year in history for number of publications – Plenty of fundamental results over last year and more coming • Experiment personpower – Resources required to operate the experiment in 2009 are well defined – MoUs provide predictions how personpower will evolve through 2009 • Success of the physics program in 2009 and beyond requires – Continuing commitment from the Collaborating groups – Strong support from the Laboratory • Summary – The Collaboration looks forward to continuing data taking in 2009 and to exploiting the exciting potential of full Run II data set June 2007 P5 Meeting at Fermilab2 The DØ Collaboration DØ is an international collaboration of 610 physicists from 19 nations who have designed, built and operate the DØ detector at the Tevatron and perform data analysis Institutions: 88 total, 38 US, 50 non-US Collaborators: ~ 50% f rom non-US i nstit uti ons (note strong European involvement) ~ 100 postdocs, 140 graduate students June 2007 P5 Meeting at Fermilab3 Physics Goals Fundamental questions Precision tests of the Standard Model Quark sub-structure? – Weak bosons, top quark, QCD, B-physics Origin of mass? Search for particles and forces beyond those known Matter-antimatter asymmetry? What is cosmic dark matter? – Higgs, supersymmetry, extra dimensions….