The Role of GCP8 in Microtubule Nucleation and Cell Cicle Progression
Total Page:16
File Type:pdf, Size:1020Kb
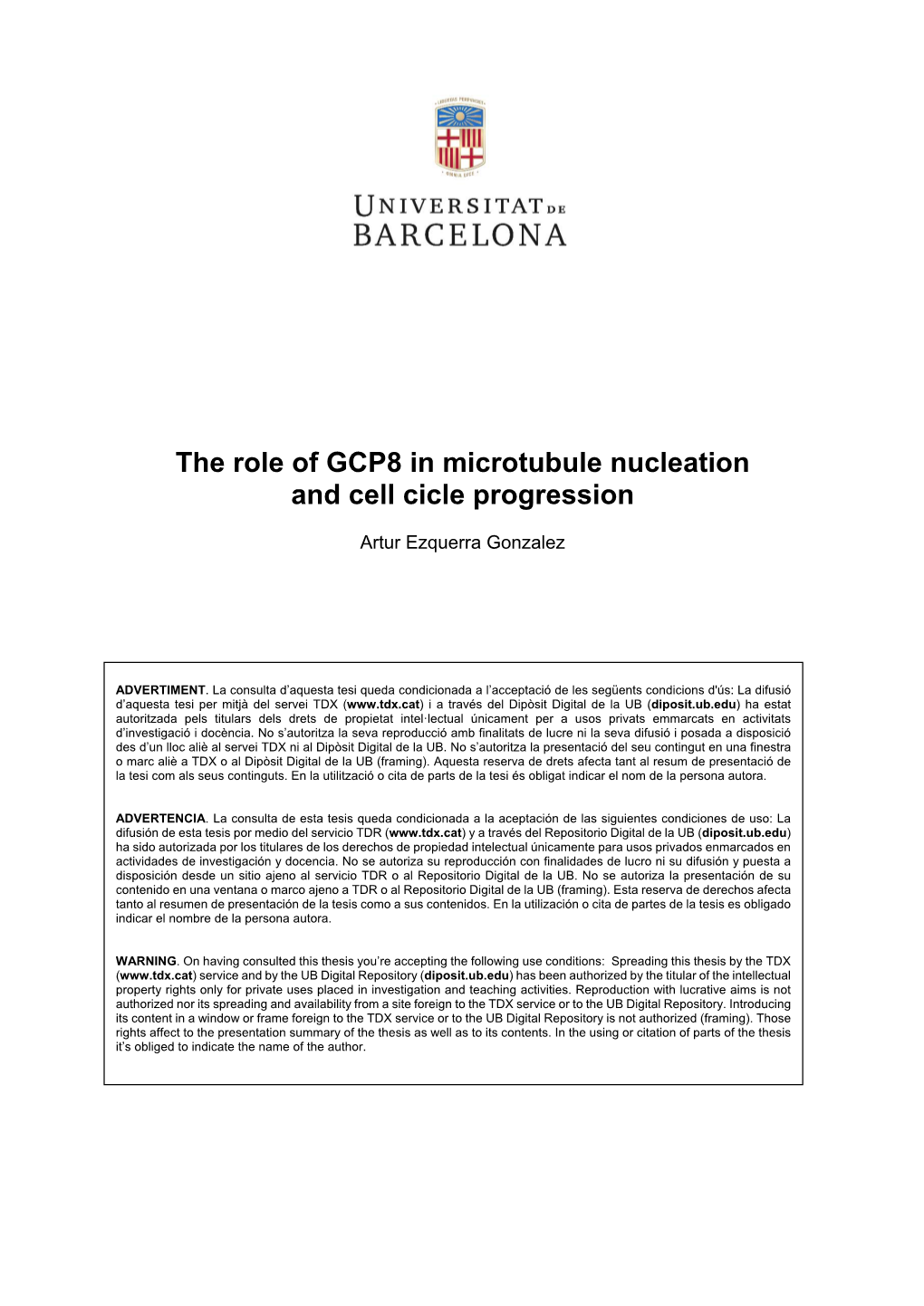
Load more
Recommended publications
-
The Ciliated Cell Transcriptome A
THE CILIATED CELL TRANSCRIPTOME A DISSERTATION SUBMITTED TO THE DEPARTMENT OF BIOLOGICAL SCIENCES AND THE COMMITTEE ON GRADUATE STUDIES OF STANFORD UNIVERSITY IN PARTIAL FULFILLMENT OF THE REQUIREMENTS FOR THE DEGREE OF DOCTOR OF PHILOSOPHY Ramona Hoh March 2010 ! © 2010 by Ramona Amy Hoh. All Rights Reserved. Re-distributed by Stanford University under license with the author. This work is licensed under a Creative Commons Attribution- Noncommercial 3.0 United States License. http://creativecommons.org/licenses/by-nc/3.0/us/ This dissertation is online at: http://purl.stanford.edu/sk794dv5857 ii I certify that I have read this dissertation and that, in my opinion, it is fully adequate in scope and quality as a dissertation for the degree of Doctor of Philosophy. Timothy Stearns, Primary Adviser I certify that I have read this dissertation and that, in my opinion, it is fully adequate in scope and quality as a dissertation for the degree of Doctor of Philosophy. Mark Krasnow I certify that I have read this dissertation and that, in my opinion, it is fully adequate in scope and quality as a dissertation for the degree of Doctor of Philosophy. Maxence Nachury I certify that I have read this dissertation and that, in my opinion, it is fully adequate in scope and quality as a dissertation for the degree of Doctor of Philosophy. William Nelson Approved for the Stanford University Committee on Graduate Studies. Patricia J. Gumport, Vice Provost Graduate Education This signature page was generated electronically upon submission of this dissertation in electronic format. An original signed hard copy of the signature page is on file in University Archives. -
Assembly of Centrosomal Proteins and Microtubule Organization Depends on PCM-1
JCBArticle Assembly of centrosomal proteins and microtubule organization depends on PCM-1 Alexander Dammermann and Andreas Merdes Wellcome Trust Centre for Cell Biology, Institute of Cell and Molecular Biology, University of Edinburgh, Edinburgh EH9 3JR, UK he protein PCM-1 localizes to cytoplasmic granules treatment of cells with the microtubule inhibitor nocodazole. known as “centriolar satellites” that are partly enriched Inhibition or depletion of PCM-1 function further disrupted T around the centrosome. We inhibited PCM-1 function the radial organization of microtubules without affecting using a variety of approaches: microinjection of antibodies microtubule nucleation. Loss of microtubule organization into cultured cells, overexpression of a PCM-1 deletion was also observed after centrin or ninein depletion. Our mutant, and specific depletion of PCM-1 by siRNA. All data suggest that PCM-1–containing centriolar satellites are Downloaded from approaches led to reduced targeting of centrin, pericentrin, involved in the microtubule- and dynactin-dependent recruit- and ninein to the centrosome. Similar effects were seen ment of proteins to the centrosome, of which centrin and upon inhibition of dynactin by dynamitin, and after prolonged ninein are required for interphase microtubule organization. jcb.rupress.org Introduction Microtubule organization is essential for directional intra- the centrosomal surface or subsequently released and anchored cellular transport, for the modulation of cell morphology in other places of the cell (Mogensen, 1999). The initial step and locomotion, and for the formation of the spindle apparatus of microtubule nucleation is dependent on the function of during cell division. With the exception of plants, most cells 25S ring complexes of the protein ␥-tubulin and associated on December 31, 2017 organize their microtubule network using specialized structures, proteins (Zheng et al., 1995). -
Specialized Cilia in Mammalian Sensory Systems
Cells 2015, 4, 500-519; doi:10.3390/cells4030500 OPEN ACCESS cells ISSN 2073-4409 www.mdpi.com/journal/cells Review Specialized Cilia in Mammalian Sensory Systems Nathalie Falk, Marlene Lösl, Nadja Schröder and Andreas Gießl * Department of Biology, Animal Physiology, University of Erlangen-Nuremberg, 91058 Erlangen, Germany; E-Mails: [email protected] (N.F.); [email protected] (M.L.); [email protected] (A.G.) * Author to whom correspondence should be addressed; E-Mail: [email protected]; Tel.: +49-9131-85-28055; Fax: +49-9131-85-28060. Academic Editors: Gang Dong and William Tsang Received: 18 May 2015 / Accepted: 9 September 2015 / Published: 11 September 2015 Abstract: Cilia and flagella are highly conserved and important microtubule-based organelles that project from the surface of eukaryotic cells and act as antennae to sense extracellular signals. Moreover, cilia have emerged as key players in numerous physiological, developmental, and sensory processes such as hearing, olfaction, and photoreception. Genetic defects in ciliary proteins responsible for cilia formation, maintenance, or function underlie a wide array of human diseases like deafness, anosmia, and retinal degeneration in sensory systems. Impairment of more than one sensory organ results in numerous syndromic ciliary disorders like the autosomal recessive genetic diseases Bardet-Biedl and Usher syndrome. Here we describe the structure and distinct functional roles of cilia in sensory organs like the inner ear, the olfactory epithelium, and the retina of the mouse. The spectrum of ciliary function in fundamental cellular processes highlights the importance of elucidating ciliopathy-related proteins in order to find novel potential therapies. -
Josephine Bay Paul Center for Comparative Molecular Biology And
OCTOBER 2, 2014 CONTACT US DIRECTIONS TO MBL HOME ABOUT MBL EDUCATION RESEARCH GIVING MBL NEWS HOME Josephine Bay Paul Center for Comparative Molecular Biology and Evolution RESOURCES HIGHLIGHTS RESEARCH Josephine Bay Paul Center for Comparative Molecular Biology and Evolution Eugene Bell Center for Regenerative Biology & Tissue Engineering Cellular Dynamics Program The Ecosystems Center Program in Sensory Physiology and Behavior Whitman Center for Research and Discovery EDUCATION MBLWHOI LIBRARY GIFTS PEOPLE In the Josephine Bay Paul Center for Comparative Molecular Biology and Evolution, scientists explore the evolution and interaction of genomes of diverse organisms with significant roles in environmental biology and human health. Bay Paul Center scientists integrate the powerful tools of genome science, molecular phylogenetics, and molecular ecology to advance our understanding of how living organisms are related to each other, to provide the tools to quantify and assess biodiversity, and to identify genes and metabolic processes of ecological and biomedical importance. Publications | Staff List © 1996-2014, The Marine Biological Laboratory MARINE BIOLOGICAL LABORATORY, MBL, and the Join the MBL community: 1888 logo are registered trademarks and service marks of The Marine Biological Laboratory. OCTOBER 2, 2014 CONTACT US DIRECTIONS TO MBL HOME ABOUT MBL EDUCATION RESEARCH GIVING MBL NEWS HOME Bay Paul Center Publications RESOURCES Akerman, NH; Butterfield, DA; and Huber, JA. 2013. Phylogenetic Diversity and Functional Gene Patterns of Sulfur- oxidizing Subseafloor Epsilonproteobacteria in Diffuse Hydrothermal Vent Fluids. Front Microbiol. 4, 185. HIGHLIGHTS RESEARCH Alliegro, MC; and Alliegro, MA. 2013. Localization of rRNA Transcribed Spacer Domains in the Nucleolinus and Maternal Procentrosomes of Surf Clam (Spisula) Oocytes.” RNA Biol. -
Centrosome Cohesion: Functions of C-NAP1
Provided by the author(s) and NUI Galway in accordance with publisher policies. Please cite the published version when available. Title Centrosome cohesion: functions of C-NAP1 Author(s) Flanagan, Anne-Marie Publication Date 2016-01-07 Item record http://hdl.handle.net/10379/5458 Downloaded 2021-09-25T13:41:15Z Some rights reserved. For more information, please see the item record link above. Centrosome Cohesion: Functions of C-NAP1 Anne-Marie Flanagan Centre for Chromosome Biology, School of Natural Sciences, National University of Ireland, Galway A thesis submitted to the National University of Ireland, Galway for the degree of Doctor of Philosophy September 2015 Supervisor: Prof. Ciaran Morrison Table of Contents Table of Contents ....................................................................................ii List of figures ........................................................................................... v List of tables ......................................................................................... viii Abreviations ............................................................................................ ix Acknowledgements ................................................................................xii Abstract ................................................................................................ xiii 1. Introduction .................................................................................... 14 1.1 Cell cycle overview .................................................................................... -
Regulation of Centriolar Satellite Integrity and Its Physiology
Cell. Mol. Life Sci. DOI 10.1007/s00018-016-2315-x Cellular and Molecular Life Sciences REVIEW Regulation of centriolar satellite integrity and its physiology 1,2 1,3 Akiko Hori • Takashi Toda Received: 19 May 2016 / Revised: 14 July 2016 / Accepted: 21 July 2016 Ó The Author(s) 2016. This article is published with open access at Springerlink.com Abstract Centriolar satellites comprise cytoplasmic gran- Keywords Cellular stress Á Centriole Á Ciliogenesis Á ules that are located around the centrosome. Their MSD1/SSX2IP Á Microtubule Á PCM1 Á PLK4 Á molecular identification was first reported more than a Phosphorylation Á Ubiquitylation quarter of a century ago. These particles are not static in the cell but instead constantly move around the centrosome. Abbreviations Over the last decade, significant advances in their molec- CS Centriolar satellites ular compositions and biological functions have been c-TuC c-Tubulin complex achieved due to comprehensive proteomics and genomics, HU Hydroxyurea super-resolution microscopy analyses and elegant genetic IR Ionising radiation manipulations. Centriolar satellites play pivotal roles in MCPH Microcephaly centrosome assembly and primary cilium formation MT Microtubule through the delivery of centriolar/centrosomal components MTOC Microtubule-organising centre from the cytoplasm to the centrosome. Their importance is PCM Pericentriolar material further underscored by the fact that mutations in genes SPB Spindle pole body encoding satellite components and regulators lead to vari- UV Ultraviolet ous human disorders such as ciliopathies. Moreover, the most recent findings highlight dynamic structural remod- elling in response to internal and external cues and unexpected positive feedback control that is exerted from Introduction the centrosome for centriolar satellite integrity. -
Gene Ontology – Biological Process
Table of Contents Abstract iv Acknowledgments vi Chapter 1: Introduction Long non-coding RNAs 1 Functions of long non-coding RNAs 4 Nuclear lncRNAs 4 Cytoplasmic lncRNAs 6 Long non-coding RNAs in cancer 9 Long non-coding RNAs in gliomas 12 Oncogenic long non-coding RNAs in gliomas 14 Tumor suppressor long non-coding RNAs in gliomas 17 References 19 Figures 33 Chapter 2: The functional role of APTR long non-coding RNA Abstract 35 Introduction 36 Results 38 Discussion 46 Materials and Methods 51 References 56 Figures 61 Chapter 3: Adapted from “LINC00152 promotes invasion in glioblastomas through a hairpin structure located near its 3’ end” My contribution to the paper 80 Abstract 82 Introduction 83 Results 84 ii Discussion 94 Materials and Methods 100 References 103 Figures 113 Chapter 4: Closing Remarks and Future Directions Alternative methods for discovering APTR interacting partners 136 APTR processing into different fragments 138 APTR and LINC00152 secondary structure 139 Identifying LINC00152 protein interactor 140 APTR as a regulator of gene expression 142 Molecular functions of LINC00152 144 Clinical potentials of APTR and LINC00152 145 References 146 Figures 152 iii Abstract Since the advent of high-throughput sequencing methods, long non-coding RNAs (lncRNAs) have been emerging as important players in physiological and pathological conditions. Among them, APTR (Alu-mediated p21 transcriptional regulator) is a lncRNA upregulated in tumors. This RNA regulates the cell cycle by recruiting the PRC2 protein complex (Polycomb Repressive Complex 2) to the promoter region of p21 gene, inducing its suppression. In addition, APTR and p21 expressions are anti-correlated in glioblastoma patient samples. -
CDK5RAP2 Functions in Centrosome to Spindle Pole Attachment and DNA Damage Response
JCB: Article CDK5RAP2 functions in centrosome to spindle pole attachment and DNA damage response Alexis R. Barr,1,2 John V. Kilmartin,3 and Fanni Gergely1,2 1Cancer Research UK Cambridge Research Institute, Cambridge CB2 0RE, England, UK 2Department of Oncology, University of Cambridge, Cambridge CB2 3RA, England, UK 3Medical Research Council Laboratory of Molecular Biology, Cambridge CB2 0QH, England, UK he centrosomal protein, CDK5RAP2, is mutated fail to recruit specific PCM components that mediate in primary microcephaly, a neurodevelopmental attachment to spindle poles. Furthermore, we show that Tdisorder characterized by reduced brain size. The the CNN1 domain enforces cohesion between parental Drosophila melanogaster homologue of CDK5RAP2, centrioles during interphase and promotes efficient DNA centrosomin (Cnn), maintains the pericentriolar matrix damage–induced G2 cell cycle arrest. Because mitotic (PCM) around centrioles during mitosis. In this study, we spindle positioning, asymmetric centrosome inheritance, demonstrate a similar role for CDK5RAP2 in vertebrate and DNA damage signaling have all been implicated cells. By disrupting two evolutionarily conserved domains in cell fate determination during neurogenesis, our find- of CDK5RAP2, CNN1 and CNN2, in the avian B cell line ings provide novel insight into how impaired CDK5RAP2 DT40, we find that both domains are essential for link- function could cause premature depletion of neural stem ing centrosomes to mitotic spindle poles. Although struc- cells and thereby microcephaly. turally intact, centrosomes lacking the CNN1 domain Introduction The centrosome consists of a pair of centrioles surrounded by 1995; Merdes et al., 1996, 2000; Gordon et al., 2001; Quintyne the pericentriolar matrix (PCM). In most animal cells, it is the and Schroer, 2002; Silk et al., 2009). -
Genome-Wide Association Study to Find Modifiers for Tetralogy of Fallot in the 22Q11.2 Deletion Syndrome Identifies Variants in the GPR98 Locus on 5Q14.3
1 2–4 * ) in an intron of −8 tetralogy of Fallot tetralogy of ◼ =2.98×10 P is the only gene that is known is the only gene that is known License, which permits use, distribution, License, which permits use, distribution, DOI: 10.1161/CIRCGENETICS.116.001690 MEF2C . Locus on 5q14.3 Data Supplement ivelo-cardio-facial syndrome ivelo-cardio-facial ◼ velocardiofacial syndrome/DiGeorge syndrome; Mendelian syndrome/DiGeorge velocardiofacial Inheritance in Man No. 192430, 188400) is one of the most births. common genomic disorders, occurring in 1 of 4000 live Over 90% of affected individuals have a de novo, hemizygous a de novo, have individuals 90% of affected Over 3 million base pair (Mb) deletion on chromosome 22q11.2. features of the syndrome, All subjects with the deletion have GPR98 1 genotype ◼ is published on behalf of the American Heart Association, Inc., by Wolters Kluwer Health, Wolters Association, Inc., by American Heart is published on behalf of the . 2017;10:e. DOI: 10.1161/CIRCGENETICS.116.001690.) Original Article (Myocyte-specific enhancer factor 2C). enhancer (Myocyte-specific Creative Commons Attribution Non-Commercial-NoDerivs Attribution Commons Creative MEF2C http://circgenetics.ahajournals.org/lookup/suppl/doi:10.1161/CIRCGENETICS.116.001690/-/DC1. DiGeorge syndrome DiGeorge Beverly S. Emanuel, PhD; Bernice E. Morrow, PhD; S. Emanuel, PhD; Bernice E. Morrow, Beverly ◼ , including (G-protein–coupled receptor V1) gene on chromosome 5q14.3. There was also suggestive evidence evidence also suggestive There was V1) gene on chromosome 5q14.3. (G-protein–coupled receptor Circ Cardiovasc Genet Circ Cardiovasc ( —To identify common genetic variants associated with TOF in individuals with 22q11.2DS, we in individuals TOF associated with identify common genetic variants —To is available at http://circgenetics.ahajournals.org is available Circulation: Cardiovascular Genetics Cardiovascular Circulation: GPR98 GPR98 chromosomes See Clinical Perspective Jonathan H. -
Machine-Learning Based Classification of Textual Stimuli To
MACHINE-LEARNING BASED CLASSIFICATION OF TEXTUAL STIMULI TO PROMOTE IDEATION IN BIOINSPIRED DESIGN A Dissertation by MICHAEL W. GLIER Submitted to the Office of Graduate Studies of Texas A&M University in partial fulfillment of the requirements for the degree of DOCTOR OF PHILOSOPHY Chair of Committee, Daniel A. McAdams Co-Chair of Committee, Julie S. Linsey Committee Members, Richard J Malak Tracy A. Hammond Head of Department, Andreas Polycarpou August 2013 Major Subject: Mechanical Engineering Copyright 2013 Michael W. Glier ABSTRACT Bioinspired design uses biological systems to inspire engineering designs. One of bioinspired design’s challenges is identifying relevant information sources in biology for an engineering design task. Currently information can be retrieved by searching biology texts or journals using biology-focused keywords that map to engineering functions. However, this search technique can overwhelm designers with unusable results. This work explores the use of text classification tools to identify relevant biology passages for design. Further, this research examines the effects of using biology passages as stimuli during idea generation. Four human-subjects studies are examined in this work. Two surveys are performed in which participants evaluate sentences from a biology corpus and indicate whether each sentence prompts an idea for solving a specific design problem. The surveys are used to develop and evaluate text classification tools. Two idea generation studies are performed in which participants generate and record solutions for designing a corn shucker using either different sets of biology passages as design stimuli, or no stimuli. Based 286 sentences from the surveys, a k Nearest Neighbor classifier is developed that is able to identify helpful sentences relating to the function “separate” with a precision of 0.62 and recall of 0.48. -
A Study of the Cell Biology of Motility in Eimeria Tenella Sporozoites
A STUDY OF THE CELL BIOLOGY OF MOTILITY IN Eimeria tenella SPOROZOITES by David Robert Bruce Department of Biology University College London A thesis presented for the degree of Doctor of Philosophy in the University of London 2000 ProQuest Number: U643145 All rights reserved INFORMATION TO ALL USERS The quality of this reproduction is dependent upon the quality of the copy submitted. In the unlikely event that the author did not send a complete manuscript and there are missing pages, these will be noted. Also, if material had to be removed, a note will indicate the deletion. uest. ProQuest U643145 Published by ProQuest LLC(2016). Copyright of the Dissertation is held by the Author. All rights reserved. This work is protected against unauthorized copying under Title 17, United States Code. Microform Edition © ProQuest LLC. ProQuest LLC 789 East Eisenhower Parkway P.O. Box 1346 Ann Arbor, Ml 48106-1346 ABSTRACT A study on the cell biology of motility inEimeria tenella sporozoites Eimeria tenella is an obligate intracellular parasite within the phylum Apicomplexa. It is the causative agent of coccidiosis in domesticated chickens and under modem farming conditions can have a considerable economic impact. Motility is employed by the sporozoite to effect release from the sporocyst and enable invasion of appropriate host cells and occurs at an average speed of 16.7 ± 6 pms'\ Frame by frame video analysis of gliding motility shows it to be an erratic non substrate specific process and this observation was confirmed by studies of bead translocation across the cell surface occurring at an average speed of 16.9 ± 7.6 pms'^ Incubation with cytochalasin D, 2,3-butanedione monoxime and colchicine, known inhibitors of the motility associated proteins actin, myosin and tubulin respectively, indicated that it is an actomyosin complex which generates the force to power sporozoite motility. -
Spatial Organization of Large Cells
Spatial Organization of Large Cells A dissertation presented by Martin Helmut Wuehr to The Committee on Higher Degrees in Systems Biology in partial fulfillment of the requirements for the degree of Doctor of Philosophy in the subject of Systems Biology Harvard University Cambridge, Massachusetts March 2010 i © 2010 – Martin H. Wuehr All rights reserved. ii Timothy John Mitchison Martin Helmut Wuehr Spatial Organization of Large Cells Abstract The rationale for my research was to investigate unusually large cells, fertilized frog and fish eggs, to obtain a unique perspective on a cell’s spatial organization with a focus on cell division. First, we investigated how spindle size changes during cleavage stages while cell size changes by orders of magnitude. To do so we improved techniques for immunofluorescence in amphibian embryos and generated a transgenic fish line with fluorescently labeled microtubules. We show that in smaller cells spindle length scales with cell length, but in very large cells spindle length approaches an upper limit and seems uncoupled from cell size. Furthermore, we were able to assemble mitotic spindles in embryonic extract that had similar size as in vivo spindles. This indicates that spindle size is set by a mechanism that is intrinsic to the spindle and not downstream of cell size. Second we investigated how relatively small spindles in large cells are positioned, and oriented for symmetric cell division. We show that the localization and orientation of these spindles are determined by location and iii orientation of sister centrosomes set by the anaphase-telophase aster of the previous cycle. Third we researched the mechanism by which asters center and align centrosomes relative to the longest axis.