Based on Space Particle Dualism Theory
Total Page:16
File Type:pdf, Size:1020Kb
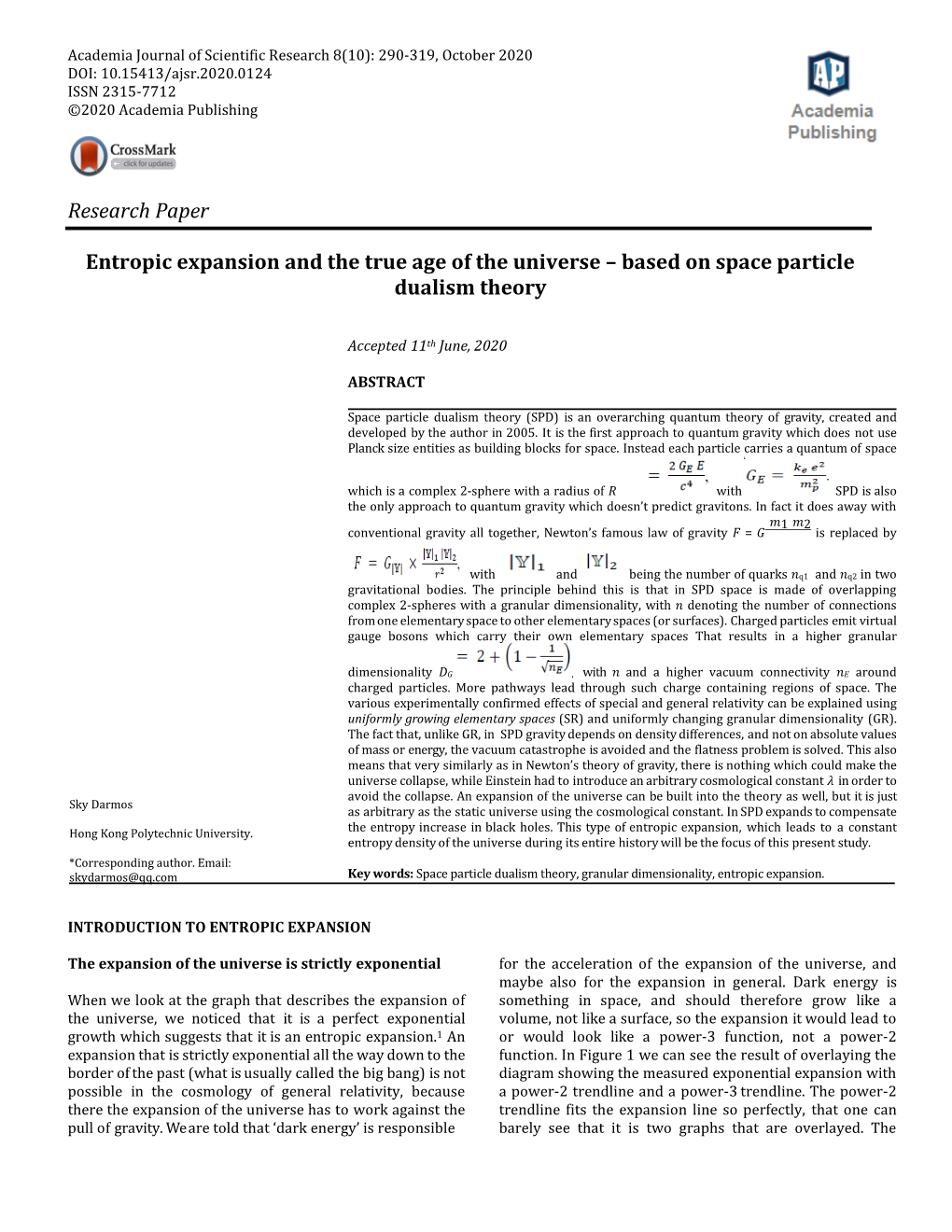
Load more
Recommended publications
-
The Global Jet Structure of the Archetypical Quasar 3C 273
galaxies Article The Global Jet Structure of the Archetypical Quasar 3C 273 Kazunori Akiyama 1,2,3,*, Keiichi Asada 4, Vincent L. Fish 2 ID , Masanori Nakamura 4, Kazuhiro Hada 3 ID , Hiroshi Nagai 3 and Colin J. Lonsdale 2 1 National Radio Astronomy Observatory, 520 Edgemont Rd, Charlottesville, VA 22903, USA 2 Massachusetts Institute of Technology, Haystack Observatory, 99 Millstone Rd, Westford, MA 01886, USA; vfi[email protected] (V.L.F.); [email protected] (C.J.L.) 3 National Astronomical Observatory of Japan, 2-21-1 Osawa, Mitaka, Tokyo 181-8588, Japan; [email protected] (K.H.); [email protected] (H.N.) 4 Institute of Astronomy and Astrophysics, Academia Sinica, P.O. Box 23-141, Taipei 10617, Taiwan; [email protected] (K.A.); [email protected] (M.N.) * Correspondence: [email protected] Received: 16 September 2017; Accepted: 8 January 2018; Published: 24 January 2018 Abstract: A key question in the formation of the relativistic jets in active galactic nuclei (AGNs) is the collimation process of their energetic plasma flow launched from the central supermassive black hole (SMBH). Recent observations of nearby low-luminosity radio galaxies exhibit a clear picture of parabolic collimation inside the Bondi accretion radius. On the other hand, little is known of the observational properties of jet collimation in more luminous quasars, where the accretion flow may be significantly different due to much higher accretion rates. In this paper, we present preliminary results of multi-frequency observations of the archetypal quasar 3C 273 with the Very Long Baseline Array (VLBA) at 1.4, 15, and 43 GHz, and Multi-Element Radio Linked Interferometer Network (MERLIN) at 1.6 GHz. -
Near-Infrared Luminosity Relations and Dust Colors L
A&A 578, A47 (2015) Astronomy DOI: 10.1051/0004-6361/201525817 & c ESO 2015 Astrophysics Obscuration in active galactic nuclei: near-infrared luminosity relations and dust colors L. Burtscher1, G. Orban de Xivry1, R. I. Davies1, A. Janssen1, D. Lutz1, D. Rosario1, A. Contursi1, R. Genzel1, J. Graciá-Carpio1, M.-Y. Lin1, A. Schnorr-Müller1, A. Sternberg2, E. Sturm1, and L. Tacconi1 1 Max-Planck-Institut für extraterrestrische Physik, Postfach 1312, Gießenbachstr., 85741 Garching, Germany e-mail: [email protected] 2 Raymond and Beverly Sackler School of Physics & Astronomy, Tel Aviv University, 69978 Ramat Aviv, Israel Received 5 February 2015 / Accepted 5 April 2015 ABSTRACT We combine two approaches to isolate the AGN luminosity at near-IR wavelengths and relate the near-IR pure AGN luminosity to other tracers of the AGN. Using integral-field spectroscopic data of an archival sample of 51 local AGNs, we estimate the fraction of non-stellar light by comparing the nuclear equivalent width of the stellar 2.3 µm CO absorption feature with the intrinsic value for each galaxy. We compare this fraction to that derived from a spectral decomposition of the integrated light in the central arcsecond and find them to be consistent with each other. Using our estimates of the near-IR AGN light, we find a strong correlation with presumably isotropic AGN tracers. We show that a significant offset exists between type 1 and type 2 sources in the sense that type 1 MIR X sources are 7 (10) times brighter in the near-IR at log LAGN = 42.5 (log LAGN = 42.5). -
Messier Objects
Messier Objects From the Stocker Astroscience Center at Florida International University Miami Florida The Messier Project Main contributors: • Daniel Puentes • Steven Revesz • Bobby Martinez Charles Messier • Gabriel Salazar • Riya Gandhi • Dr. James Webb – Director, Stocker Astroscience center • All images reduced and combined using MIRA image processing software. (Mirametrics) What are Messier Objects? • Messier objects are a list of astronomical sources compiled by Charles Messier, an 18th and early 19th century astronomer. He created a list of distracting objects to avoid while comet hunting. This list now contains over 110 objects, many of which are the most famous astronomical bodies known. The list contains planetary nebula, star clusters, and other galaxies. - Bobby Martinez The Telescope The telescope used to take these images is an Astronomical Consultants and Equipment (ACE) 24- inch (0.61-meter) Ritchey-Chretien reflecting telescope. It has a focal ratio of F6.2 and is supported on a structure independent of the building that houses it. It is equipped with a Finger Lakes 1kx1k CCD camera cooled to -30o C at the Cassegrain focus. It is equipped with dual filter wheels, the first containing UBVRI scientific filters and the second RGBL color filters. Messier 1 Found 6,500 light years away in the constellation of Taurus, the Crab Nebula (known as M1) is a supernova remnant. The original supernova that formed the crab nebula was observed by Chinese, Japanese and Arab astronomers in 1054 AD as an incredibly bright “Guest star” which was visible for over twenty-two months. The supernova that produced the Crab Nebula is thought to have been an evolved star roughly ten times more massive than the Sun. -
Hot Interstellar Matter in Elliptical Galaxies
Hot Interstellar Matter in Elliptical Galaxies For further volumes: http://www.springer.com/series/5664 Astrophysics and Space Science Library EDITORIAL BOARD Chairman W. B. BURTON, National Radio Astronomy Observatory, Charlottesville, Virginia, U.S.A. ([email protected]); University of Leiden, The Netherlands ([email protected]) F. BERTOLA, University of Padua, Italy J. P. CASSINELLI, University of Wisconsin, Madison, U.S.A. C. J. CESARSKY, Commission for Atomic Energy, Saclay, France P. EHRENFREUND, Leiden University, The Netherlands O. ENGVOLD, University of Oslo, Norway A. HECK, Strasbourg Astronomical Observatory, France E. P. J. VAN DEN HEUVEL, University of Amsterdam, The Netherlands V. M. KASPI, McGill University, Montreal, Canada J. M. E. KUIJPERS, University of Nijmegen, The Netherlands H. VAN DER LAAN, University of Utrecht, The Netherlands P. G. MURDIN, Institute of Astronomy, Cambridge, UK F. PACINI, Istituto Astronomia Arcetri, Firenze, Italy V. RADHAKRISHNAN, Raman Research Institute, Bangalore, India B . V. S O M OV, Astronomical Institute, Moscow State University, Russia R. A. SUNYAEV, Space Research Institute, Moscow, Russia Dong-Woo Kim Silvia Pellegrini Editors Hot Interstellar Matter in Elliptical Galaxies 123 Editors Dong-Woo Kim Harvard Smithsonian Center for Astrophysics Garden Street 60 02138 Cambridge Massachusetts USA [email protected] Silvia Pellegrini Dipartimento di Astronomia Universita` di Bologna Via Ranzani 1 40127 Bologna Italy [email protected] Cover figure: Chandra image of NGC 7619. From Kim et al. (2008). Reproduced by permission of the AAS. ISSN 0067-0057 ISBN 978-1-4614-0579-5 e-ISBN 978-1-4614-0580-1 DOI 10.1007/978-1-4614-0580-1 Springer Heidelberg Dordrecht London New York Library of Congress Control Number: 2011938147 c Springer Science+Business Media, LLC 2012 This work is subject to copyright. -
Star Maps: Where Are the Black Holes?
BLACK HOLE FAQ’s 1. What is a black hole? A black hole is a region of space that has so much mass concentrated in it that there is no way for a nearby object to escape its gravitational pull. There are three kinds of black hole that we have strong evidence for: a. Stellar-mass black holes are the remaining cores of massive stars after they die in a supernova explosion. b. Mid-mass black hole in the centers of dense star clusters Credit : ESA, NASA, and F. Mirabel c. Supermassive black hole are found in the centers of many (and maybe all) galaxies. 2. Can a black hole appear anywhere? No, you need an amount of matter more than 3 times the mass of the Sun before it can collapse to create a black hole. 3. If a star dies, does it always turn into a black hole? No, smaller stars like our Sun end their lives as dense hot stars called white dwarfs. Much more massive stars end their lives in a supernova explosion. The remaining cores of only the most massive stars will form black holes. 4. Will black holes suck up all the matter in the universe? No. A black hole has a very small region around it from which you can't escape, called the “event horizon”. If you (or other matter) cross the horizon, you will be pulled in. But as long as you stay outside of the horizon, you can avoid getting pulled in if you are orbiting fast enough. 5. What happens when a spaceship you are riding in falls into a black hole? Your spaceship, along with you, would be squeezed and stretched until it was torn completely apart as it approached the center of the black hole. -
Arxiv:2007.09714V1 [Gr-Qc] 19 Jul 2020 Tem PSR J0737-3039A/B
Probing Noncommutative Gravity with Gravitational Wave and Binary Pulsar Observations Leah Jenks,1 Kent Yagi,2 and Stephon Alexander1 1Brown Theoretical Physics Center and Department of Physics, Brown University, 182 Hope Street, Providence, Rhode Island, 02903 2Department of Physics, University of Virginia, P.O. Box 400714, Charlottesville, VA 22904-4714, USA (Dated: July 21, 2020) Noncommutative gravity is a natural method of quantizing spacetime by promoting the spacetime coordinates themselves to operators which do not commute. This approach is motivated, for exam- ple, from a quantum gravity perspective, among others. Noncommutative gravity has been tested against the binary black hole merger event GW150914. Here, we extend and improve upon such a previous analysis by (i) relaxing an assumption made on the preferred direction due to noncommuta- tivity, (ii) using posterior samples produced by the LIGO/Virgo Collaborations, (iii) consider other gravitational wave events, namely GW151226, GW170608, GW170814 and GW170817, and (iv) consider binary pulsar observations. Using Kepler's law that contains the noncommutative effect at second post-Newtonian order, we derive corrections to the gravitational waveform phase and the pericenter precession. Using the gravitational wave and double pulsar binary observations, we find bounds on a space-time noncommutative tensor θ0i in terms of the preferred frame direction with respect to the orientation of each binary. We find that the gravitational wave bounds are stronger than the binary pulsar one by an order of magnitude and the noncommutative tensor normalized by the Planck length and time is constrained to be of order unity. I. INTRODUCTION of noncommutative gravity stems from these theories. -
Cinemática Estelar, Modelos Dinâmicos E Determinaç˜Ao De
UNIVERSIDADE FEDERAL DO RIO GRANDE DO SUL PROGRAMA DE POS´ GRADUA¸CAO~ EM F´ISICA Cinem´atica Estelar, Modelos Din^amicos e Determina¸c~ao de Massas de Buracos Negros Supermassivos Daniel Alf Drehmer Tese realizada sob orienta¸c~aoda Professora Dra. Thaisa Storchi Bergmann e apresen- tada ao Programa de P´os-Gradua¸c~aoem F´ısica do Instituto de F´ısica da UFRGS em preenchimento parcial dos requisitos para a obten¸c~aodo t´ıtulo de Doutor em Ci^encias. Porto Alegre Mar¸co, 2015 Agradecimentos Gostaria de agradecer a todas as pessoas que de alguma forma contribu´ıram para a realiza¸c~ao deste trabalho e em especial agrade¸co: • A` Profa. Dra. Thaisa Storchi-Bergmann pela competente orienta¸c~ao. • Aos professores Dr. Fabr´ıcio Ferrari da Universidade Federal do Rio Grande, Dr. Rogemar Riffel da Universidade Federal de Santa Maria e ao Dr. Michele Cappellari da Universidade de Oxford cujas colabora¸c~oes foram fundamentais para a realiza¸c~ao deste trabalho. • A todos os professores do Departamento de Astronomia do Instituto de F´ısica da Universidade Federal do Rio Grande do Sul que contribu´ıram para minha forma¸c~ao. • Ao CNPq pelo financiamento desse trabalho. • Aos meus pais Ingon e Selia e meus irm~aos Neimar e Carla pelo apoio. Daniel Alf Drehmer Universidade Federal do Rio Grande do Sul Mar¸co2015 i Resumo O foco deste trabalho ´eestudar a influ^encia de buracos negros supermassivos (BNSs) nucleares na din^amica e na cinem´atica estelar da regi~ao central das gal´axias e determinar a massa destes BNSs. -
Virgo the Virgin
Virgo the Virgin Virgo is one of the constellations of the zodiac, the group tion Virgo itself. There is also the connection here with of 12 constellations that lies on the ecliptic plane defined “The Scales of Justice” and the sign Libra which lies next by the planets orbital orientation around the Sun. Virgo is to Virgo in the Zodiac. The study of astronomy had a one of the original 48 constellations charted by Ptolemy. practical “time keeping” aspect in the cultures of ancient It is the largest constellation of the Zodiac and the sec- history and as the stars of Virgo appeared before sunrise ond - largest constellation after Hydra. Virgo is bordered by late in the northern summer, many cultures linked this the constellations of Bootes, Coma Berenices, Leo, Crater, asterism with crops, harvest and fecundity. Corvus, Hydra, Libra and Serpens Caput. The constella- tion of Virgo is highly populated with galaxies and there Virgo is usually depicted with angel - like wings, with an are several galaxy clusters located within its boundaries, ear of wheat in her left hand, marked by the bright star each of which is home to hundreds or even thousands of Spica, which is Latin for “ear of grain”, and a tall blade of galaxies. The accepted abbreviation when enumerating grass, or a palm frond, in her right hand. Spica will be objects within the constellation is Vir, the genitive form is important for us in navigating Virgo in the modern night Virginis and meteor showers that appear to originate from sky. Spica was most likely the star that helped the Greek Virgo are called Virginids. -
And Ecclesiastical Cosmology
GSJ: VOLUME 6, ISSUE 3, MARCH 2018 101 GSJ: Volume 6, Issue 3, March 2018, Online: ISSN 2320-9186 www.globalscientificjournal.com DEMOLITION HUBBLE'S LAW, BIG BANG THE BASIS OF "MODERN" AND ECCLESIASTICAL COSMOLOGY Author: Weitter Duckss (Slavko Sedic) Zadar Croatia Pусскй Croatian „If two objects are represented by ball bearings and space-time by the stretching of a rubber sheet, the Doppler effect is caused by the rolling of ball bearings over the rubber sheet in order to achieve a particular motion. A cosmological red shift occurs when ball bearings get stuck on the sheet, which is stretched.“ Wikipedia OK, let's check that on our local group of galaxies (the table from my article „Where did the blue spectral shift inside the universe come from?“) galaxies, local groups Redshift km/s Blueshift km/s Sextans B (4.44 ± 0.23 Mly) 300 ± 0 Sextans A 324 ± 2 NGC 3109 403 ± 1 Tucana Dwarf 130 ± ? Leo I 285 ± 2 NGC 6822 -57 ± 2 Andromeda Galaxy -301 ± 1 Leo II (about 690,000 ly) 79 ± 1 Phoenix Dwarf 60 ± 30 SagDIG -79 ± 1 Aquarius Dwarf -141 ± 2 Wolf–Lundmark–Melotte -122 ± 2 Pisces Dwarf -287 ± 0 Antlia Dwarf 362 ± 0 Leo A 0.000067 (z) Pegasus Dwarf Spheroidal -354 ± 3 IC 10 -348 ± 1 NGC 185 -202 ± 3 Canes Venatici I ~ 31 GSJ© 2018 www.globalscientificjournal.com GSJ: VOLUME 6, ISSUE 3, MARCH 2018 102 Andromeda III -351 ± 9 Andromeda II -188 ± 3 Triangulum Galaxy -179 ± 3 Messier 110 -241 ± 3 NGC 147 (2.53 ± 0.11 Mly) -193 ± 3 Small Magellanic Cloud 0.000527 Large Magellanic Cloud - - M32 -200 ± 6 NGC 205 -241 ± 3 IC 1613 -234 ± 1 Carina Dwarf 230 ± 60 Sextans Dwarf 224 ± 2 Ursa Minor Dwarf (200 ± 30 kly) -247 ± 1 Draco Dwarf -292 ± 21 Cassiopeia Dwarf -307 ± 2 Ursa Major II Dwarf - 116 Leo IV 130 Leo V ( 585 kly) 173 Leo T -60 Bootes II -120 Pegasus Dwarf -183 ± 0 Sculptor Dwarf 110 ± 1 Etc. -
2014 Observers Challenge List
2014 TMSP Observer's Challenge Atlas page #s # Object Object Type Common Name RA, DEC Const Mag Mag.2 Size Sep. U2000 PSA 18h31m25s 1 IC 1287 Bright Nebula Scutum 20'.0 295 67 -10°47'45" 18h31m25s SAO 161569 Double Star 5.77 9.31 12.3” -10°47'45" Near center of IC 1287 18h33m28s NGC 6649 Open Cluster 8.9m Integrated 5' -10°24'10" Can be seen in 3/4d FOV with above. Brightest star is 13.2m. Approx 50 stars visible in Binos 18h28m 2 NGC 6633 Open Cluster Ophiuchus 4.6m integrated 27' 205 65 Visible in Binos and is about the size of a full Moon, brightest star is 7.6m +06°34' 17h46m18s 2x diameter of a full Moon. Try to view this cluster with your naked eye, binos, and a small scope. 3 IC 4665 Open Cluster Ophiuchus 4.2m Integrated 60' 203 65 +05º 43' Also check out “Tweedle-dee and Tweedle-dum to the east (IC 4756 and NGC 6633) A loose open cluster with a faint concentration of stars in a rich field, contains about 15-20 stars. 19h53m27s Brightest star is 9.8m, 5 stars 9-11m, remainder about 12-13m. This is a challenge obJect to 4 Harvard 20 Open Cluster Sagitta 7.7m integrated 6' 162 64 +18°19'12" improve your observation skills. Can you locate the miniature coathanger close by at 19h 37m 27s +19d? Constellation star Corona 5 Corona Borealis 55 Trace the 7 stars making up this constellation, observe and list the colors of each star asterism Borealis 15H 32' 55” Theta Corona Borealis Double Star 4.2m 6.6m .97” 55 Theta requires about 200x +31° 21' 32” The direction our Sun travels in our galaxy. -
Perseus Perseus
Lateinischer Name: Deutscher Name: Per Perseus Perseus Atlas (2000.0) Karte Cambridge Star Kulmination um 2, 3 Atlas Mitternacht: Benachbarte 1, 4, Sky Atlas Sternbilder: 5 And Ari Aur Cam Cas 7. November Tau Tri Deklinationsbereich: 30° ... 59° Fläche am Himmel: 615° 2 Mythologie und Geschichte: Akrisios war der König von Argos und hatte eine hübsche Tochter namens Danae. Das Orakel von Delphi prophezeite ihm, sein Enkelsohn werde ihn einst töten. Aus Furcht vor diesem Orakelspruch ließ Akrisios seine Tochter Danae in einen Turm einschließen, damit sie keinen Mann empfangen könne. Akrisios konnte natürlich nicht wissen, dass Zeus selbst sich der liebreizenden Danae nähern wollte. Zeus tat dies auch und nahm dazu die Form eines goldenen Regens an, der durch die Wände, Fugen, Ritzen und Fenster drang. Danae gebar einen Sohn, welcher "Der aus fließendem Gold entstandene" oder auch "Der Goldgeborene" genannt wurde - eben den Perseus. Akrisios blieb dies natürlich nicht lange verborgen und er ließ seine Tochter und sein Enkelkind in einen Kasten einnageln, der dann ins Meer geworfen wurde. Danae und Perseus trieben lange Zeit auf dem Meer, bis sie schließlich an der Küste der steinigen Insel Seriphos strandeten und aus ihrem Gefängnis befreit wurden. Auf dieser Insel herrschte Polydektes, der bald jahrelang um die schöne Danae warb, jedoch vergebens. Entweder um Danae doch noch zur Ehe zu zwingen oder um Perseus aus dem Weg zu schaffen, schickte er den inzwischen zum Jüngling herangewachsenen Sohn der Danae aus, eine tödliche Aufgabe zu erledigen. Er solle das Haupt der Medusa zu holen. Die Medusa war eine der Gorgonen, eine der drei Töchter des Phorkys, die mit Schlangenhaaren ausgestattet waren und einen versteinernden Blick werfen konnten. -
Supernova Physics with Gravitational Waves: Newborn Black Holes Are “Kicked”
Supernova physics with gravitational waves: Newborn black holes are “kicked” Richard O’Shaughnessy [email protected] 614 906 9649 Davide Gerosa [email protected] 626 395 6829 Daniel Wysocki [email protected] ! ! Accepted for publication in Physical Review Letters Poster 317.07 [see iPoster] June 5, AAS 2 3 GW151226: Gravitational waves from a black hole binary B. P.• ABBOTTGW151226et al. is the second, less massive binary black hole confidently detectedPHYS. by REV. LIGO X 6, 041015 (2016) GW151226 Abbott et al, PRX 6, 041015 (2016) ; PRL 118 221101 (2017) FIG. 4. Posterior probability densities of the masses, spins, and distance to the three events GW150914, LVT151012, and GW151226. source For the two-dimensional distributions, the contours show 50% and 90% credible regions. Top left panel: Component masses m1 and source source source m2 for the three events. We use the convention that m1 ≥ m2 , which produces the sharp cut in the two-dimensional source 0.3 distribution. For GW151226 and LVT151012, the contours follow lines of constant chirp mass (M 8.9−þ0.3 M and source 1.4 ¼ ⊙ M 15:1−þ1.1 M , respectively). In all three cases, both masses are consistent with being black holes. Top right panel: The mass and¼ dimensionless⊙ spin magnitude of the final black holes. Bottom left panel: The effective spin and mass ratios of the binary components. Bottom right panel: The luminosity distance to the three events. following section and are consistent with our expect- closely mirror the original analysis of GW150914, as ations for an astrophysical BBH source.