University of Florida Thesis Or Dissertation
Total Page:16
File Type:pdf, Size:1020Kb
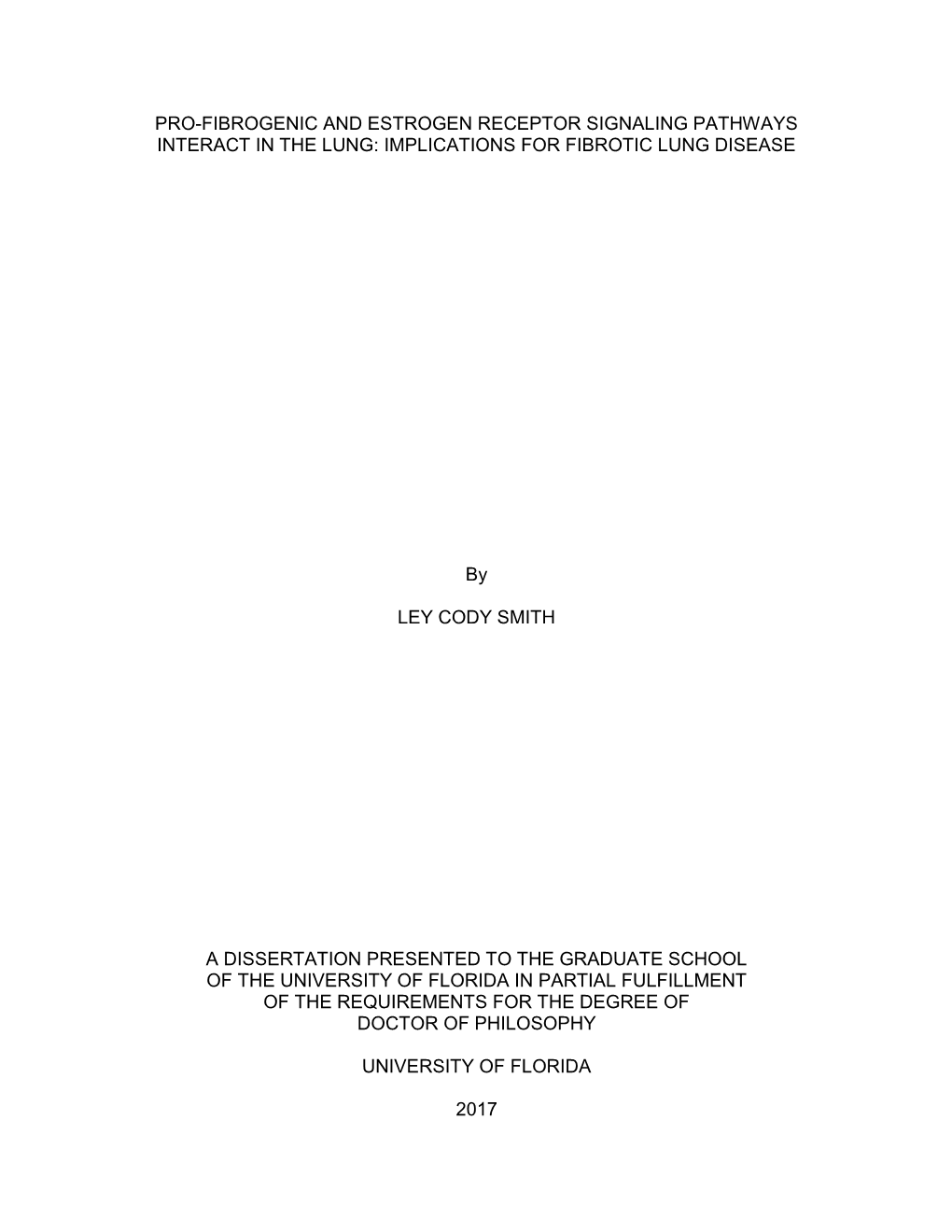
Load more
Recommended publications
-
KLF2 Induced
UvA-DARE (Digital Academic Repository) The transcription factor KLF2 in vascular biology Boon, R.A. Publication date 2008 Link to publication Citation for published version (APA): Boon, R. A. (2008). The transcription factor KLF2 in vascular biology. General rights It is not permitted to download or to forward/distribute the text or part of it without the consent of the author(s) and/or copyright holder(s), other than for strictly personal, individual use, unless the work is under an open content license (like Creative Commons). Disclaimer/Complaints regulations If you believe that digital publication of certain material infringes any of your rights or (privacy) interests, please let the Library know, stating your reasons. In case of a legitimate complaint, the Library will make the material inaccessible and/or remove it from the website. Please Ask the Library: https://uba.uva.nl/en/contact, or a letter to: Library of the University of Amsterdam, Secretariat, Singel 425, 1012 WP Amsterdam, The Netherlands. You will be contacted as soon as possible. UvA-DARE is a service provided by the library of the University of Amsterdam (https://dare.uva.nl) Download date:23 Sep 2021 Supplementary data: Genes induced by KLF2 Dekker et al. LocusLink Accession Gene Sequence Description Fold p-value ID number symbol change (FDR) 6654 AK022099 SOS1 cDNA FLJ12037 fis, clone HEMBB1001921. 100.00 5.9E-09 56999 AF086069 ADAMTS9 full length insert cDNA clone YZ35C05. 100.00 1.2E-09 6672 AF085934 SP100 full length insert cDNA clone YR57D07. 100.00 6.7E-13 9031 AF132602 BAZ1B Williams Syndrome critical region WS25 mRNA, partial sequence. -
Transcriptional Regulatory Networks in Neocortical Development
Review From trans to cis: transcriptional regulatory networks in neocortical development 1 1 1,2 Mikihito Shibata , Forrest O. Gulden , and Nenad Sestan 1 Department of Neurobiology and Kavli Institute for Neuroscience, Yale School of Medicine, New Haven, CT 06510, USA 2 Department of Psychiatry and Program in Cellular Neuroscience, Neurodegeneration and Repair, Yale School of Medicine, New Haven, CT 06510, USA Transcriptional mechanisms mediated by the binding of tions among TFs and CREs form the central core of tran- transcription factors (TFs) to cis-acting regulatory ele- scriptional regulatory networks (TRNs) (Box 1) [1]. ments (CREs) in DNA play crucial roles in directing gene Interactions between TFs and CREs are complex, dynam- expression. While TFs have been extensively studied, ic,andmodulatedbyvariousepigeneticprocesses[12].While less effort has gone towards the identification and func- individual CREs may be regulated by the competitive or tional characterization of CREs and associated epigenet- cooperative binding of many TFs, multiple CREs may also ic modulation. However, owing to methodological and confer transcriptional control over the expression of any analytical advances, more comprehensive studies of single gene [12]. Furthermore, TFs and CREs regulate regulatory elements and mechanisms are now possible. transcription together with their cognate transcriptional We summarize recent progress in integrative analyses of cofactors, chromatin regulators, epigenetic modifications, these regulatory components in the development of the RNA-binding proteins, and non-coding RNAs [2,13]. This cerebral neocortex, the part of the brain involved in combinatorial and multi-level transcriptional regulation cognition and complex behavior. These studies are greatly increases the complexity of gene expression regula- uncovering not only the underlying transcriptional reg- tion, facilitating the unique spatio-temporal patterns nec- ulatory networks, but also how these networks are essary for tissue development and function [3]. -
Consideration of Layer-Specific Marker Expression
Cerebral Cortex March 2011;21:588--596 doi:10.1093/cercor/bhq125 Advance Access publication July 12, 2010 Neocortical Layer Formation of Human Developing Brains and Lissencephalies: Consideration of Layer-Specific Marker Expression Takashi Saito1,2, Sae Hanai1,2, Sachio Takashima3, Eiji Nakagawa2, Shin Okazaki4, Takeshi Inoue5, Rie Miyata6, Kyoko Hoshino7, Takumi Akashi8, Masayuki Sasaki2, Yu-ichi Goto1, Masaharu Hayashi6 and Masayuki Itoh1 1Department of Mental Retardation and Birth Defect Research, National Center of Neurology and Psychiatry, Kodaira, 187-8502, Japan, 2Department of Child Neurology, The Hospital of National Center of Neurology and Psychiatry, Kodaira, 187-8551, Japan, 3Yanagawa Institute of Handicapped Children, International University of Health and Welfare, Fukuoka, 832-0058, Japan, 4Department of Pediatrics, Osaka City General Hospital, Osaka, 534-0021, Japan, 5Department of Pathology and Laboratory Medicine, Osaka City General Hospital, Osaka, 534-0021, Japan, 6Department of Clinical Neuropathology, Tokyo Metropolitan Institute of Neuroscience, Fuchu, 183-8526, Japan, 7Department of Pediatrics, Saitama Medical Center, Kawagoe, 350-8550, Japan and 8Department of Pathology and Laboratory Medicine, Tokyo Medical and Dental University, Tokyo, 113-8510, Japan Takashi Saito and Masayuki Itoh have contributed equally to this work. Downloaded from Address correspondence to M Itoh, Department of Mental Retardation and Birth Defect Research, National Center of Neurology and Psychiatry, 4-1-1 Ogawahigashi, Kodaira, Tokyo 187-8502, Japan. Email: [email protected]. To investigate layer-specific molecule expression in human de- each layer has a specific function with a synaptic connection. veloping neocortices, we performed immunohistochemistry of the In each step, specific genes have important roles, and the http://cercor.oxfordjournals.org/ layer-specific markers (TBR1, FOXP1, SATB2, OTX1, CUTL1, and molecular mechanism is well known in rodent brains (Arlotta CTIP2), using frontal neocortices of the dorsolateral precentral gyri et al. -
Integrative Bulk and Single-Cell Profiling of Premanufacture T-Cell Populations Reveals Factors Mediating Long-Term Persistence of CAR T-Cell Therapy
Published OnlineFirst April 5, 2021; DOI: 10.1158/2159-8290.CD-20-1677 RESEARCH ARTICLE Integrative Bulk and Single-Cell Profiling of Premanufacture T-cell Populations Reveals Factors Mediating Long-Term Persistence of CAR T-cell Therapy Gregory M. Chen1, Changya Chen2,3, Rajat K. Das2, Peng Gao2, Chia-Hui Chen2, Shovik Bandyopadhyay4, Yang-Yang Ding2,5, Yasin Uzun2,3, Wenbao Yu2, Qin Zhu1, Regina M. Myers2, Stephan A. Grupp2,5, David M. Barrett2,5, and Kai Tan2,3,5 Downloaded from cancerdiscovery.aacrjournals.org on October 1, 2021. © 2021 American Association for Cancer Research. Published OnlineFirst April 5, 2021; DOI: 10.1158/2159-8290.CD-20-1677 ABSTRACT The adoptive transfer of chimeric antigen receptor (CAR) T cells represents a breakthrough in clinical oncology, yet both between- and within-patient differences in autologously derived T cells are a major contributor to therapy failure. To interrogate the molecular determinants of clinical CAR T-cell persistence, we extensively characterized the premanufacture T cells of 71 patients with B-cell malignancies on trial to receive anti-CD19 CAR T-cell therapy. We performed RNA-sequencing analysis on sorted T-cell subsets from all 71 patients, followed by paired Cellular Indexing of Transcriptomes and Epitopes (CITE) sequencing and single-cell assay for transposase-accessible chromatin sequencing (scATAC-seq) on T cells from six of these patients. We found that chronic IFN signaling regulated by IRF7 was associated with poor CAR T-cell persistence across T-cell subsets, and that the TCF7 regulon not only associates with the favorable naïve T-cell state, but is maintained in effector T cells among patients with long-term CAR T-cell persistence. -
Free Fatty Acids Rewire Cancer Metabolism in Obesity-Associated
Published OnlineFirst March 12, 2019; DOI: 10.1158/0008-5472.CAN-18-2849 Cancer Metabolism and Chemical Biology Research Free Fatty Acids Rewire Cancer Metabolism in Obesity-Associated Breast Cancer via Estrogen Receptor and mTOR Signaling Zeynep Madak-Erdogan1,2,3,4,5,6, Shoham Band1,YiruC.Zhao1, Brandi P. Smith1, Eylem Kulkoyluoglu-Cotul1, Qianying Zuo1, Ashlie Santaliz Casiano2, Kinga Wrobel1, Gianluigi Rossi7, Rebecca L. Smith7, Sung Hoon Kim8, John A. Katzenellenbogen8, Mariah L. Johnson9, Meera Patel9, Natascia Marino9,10, Anna Maria V. Storniolo9,10, and Jodi A. Flaws11 Abstract Obesity is a risk factor for postmenopausal estrogen recep- naling, was able to block changes induced by FFA and was þ tor alpha (ERa)-positive (ER ) breast cancer. Molecular more effective in the presence of FFA. Collectively, these data mechanisms underlying factors from plasma that contribute suggest a role for obesity-associated gene and metabolic rewir- þ to this risk and how these mechanisms affect ERa signaling ing in providing new targetable vulnerabilities for ER breast have yet to be elucidated. To identify such mechanisms, we cancer in postmenopausal women. Furthermore, they provide performed whole metabolite and protein profiling in plasma a basis for preclinical and clinical trials where the impact of samples from women at high risk for breast cancer, which led agents that target ERa and mTOR signaling cross-talk would be þ us to focus on factors that were differentially present in plasma tested to prevent ER breast cancers in obese postmenopausal of obese versus nonobese postmenopausal women. These women. studies, combined with in vitro assays, identified free fatty acids (FFA) as circulating plasma factors that correlated with Significance: These findings show that obesity-associated þ increased proliferation and aggressiveness in ER breast cancer changes in certain blood metabolites rewire metabolic pro- cells. -
An Overview of the Main Genetic, Epigenetic and Environmental Factors Involved in Autism Spectrum Disorder Focusing on Synaptic Activity
International Journal of Molecular Sciences Review An Overview of the Main Genetic, Epigenetic and Environmental Factors Involved in Autism Spectrum Disorder Focusing on Synaptic Activity 1, 1, 1 2 Elena Masini y, Eleonora Loi y, Ana Florencia Vega-Benedetti , Marinella Carta , Giuseppe Doneddu 3, Roberta Fadda 4 and Patrizia Zavattari 1,* 1 Department of Biomedical Sciences, Unit of Biology and Genetics, University of Cagliari, 09042 Cagliari, Italy; [email protected] (E.M.); [email protected] (E.L.); [email protected] (A.F.V.-B.) 2 Center for Pervasive Developmental Disorders, Azienda Ospedaliera Brotzu, 09121 Cagliari, Italy; [email protected] 3 Centro per l’Autismo e Disturbi correlati (CADc), Nuovo Centro Fisioterapico Sardo, 09131 Cagliari, Italy; [email protected] 4 Department of Pedagogy, Psychology, Philosophy, University of Cagliari, 09123 Cagliari, Italy; [email protected] * Correspondence: [email protected] The authors contributed equally to this work. y Received: 30 September 2020; Accepted: 30 October 2020; Published: 5 November 2020 Abstract: Autism spectrum disorder (ASD) is a neurodevelopmental disorder that affects social interaction and communication, with restricted interests, activity and behaviors. ASD is highly familial, indicating that genetic background strongly contributes to the development of this condition. However, only a fraction of the total number of genes thought to be associated with the condition have been discovered. Moreover, other factors may play an important role in ASD onset. In fact, it has been shown that parental conditions and in utero and perinatal factors may contribute to ASD etiology. More recently, epigenetic changes, including DNA methylation and micro RNA alterations, have been associated with ASD and proposed as potential biomarkers. -
Haploinsufficiency of Autism Causative Gene Tbr1 Impairs Olfactory
Huang et al. Molecular Autism (2019) 10:5 https://doi.org/10.1186/s13229-019-0257-5 RESEARCH Open Access Haploinsufficiency of autism causative gene Tbr1 impairs olfactory discrimination and neuronal activation of the olfactory system in mice Tzyy-Nan Huang1†, Tzu-Li Yen1†, Lily R. Qiu2, Hsiu-Chun Chuang1,4, Jason P. Lerch2,3 and Yi-Ping Hsueh1* Abstract Background: Autism spectrum disorders (ASD) exhibit two clusters of core symptoms, i.e., social and communication impairment, and repetitive behaviors and sensory abnormalities. Our previous study demonstrated that TBR1, a causative gene of ASD, controls axonal projection and neuronal activation of amygdala and regulates social interaction and vocal communication in a mouse model. Behavioral defects caused by Tbr1 haploinsufficiency can be ameliorated by increasing neural activity via D-cycloserine treatment, an N-methyl-D-aspartate receptor (NMDAR) coagonist. In this report,weinvestigatetheroleofTBR1inregulatingolfaction and test whether D-cycloserine can also improve olfactory defects in Tbr1 mutant mice. Methods: We used Tbr1+/− mice as a model to investigate the function of TBR1 in olfactory sensation and discrimination of non-social odors. We employed a behavioral assay to characterize the olfactory defects of Tbr1+/− mice. Magnetic resonance imaging (MRI) and histological analysis were applied to characterize anatomical features. Immunostaining was performed to further analyze differences in expression of TBR1 subfamily members (namely TBR1, TBR2, and TBX21), interneuron populations, and dendritic abnormalities in olfactory bulbs. Finally, C-FOS staining was used to monitor neuronal activation of the olfactory system upon odor stimulation. Results: Tbr1+/− mice exhibited smaller olfactory bulbs and anterior commissures, reduced interneuron populations, and an abnormal dendritic morphology of mitral cells in the olfactory bulbs. -
PIIS0092867412011865.Pdf
918 1 Cell Cell SnapShot: Cortical Development 151 ??? SnapShot:Mollie B. Woodworth,1,2,5 Luciano XXXXX Custo Greig,1,2,5 ArnoldX R.XX Kriegstein,XX3,4 andXX JeffreyXX D. MacklisXX1,2 XXXXXXXXXX , November 9,2012©2012 ElsevierInc. 10.1016/j.cell.2012.10.004 DOI http://dx.doi.org/ , ??MONTH?? ??DATE??, 200? ©200? Elsevier Inc. 200?©200? ElsevierInc. , ??MONTH?? ??DATE??, DOI AUTHOR1Department XXXXXXXXXXXXXXXXXXXXXXXXXXXXXXXXXXXXXX of Stem Cell and Regenerative Biology and Harvard Stem Cell Institute, Harvard University, Cambridge, MA 02138, USA; 2Harvard AFFILIATIONMedical School, XXXXXXXXXXXXXXXXXXXXXXXXXXXXXXXXXXXXXXXXXXXXXXXXXXXXXXXXXXXXXXXXXXXXXXXXXXXXXXXXXXXXXXXXXXX Boston, MA 02115, USA; 3Eli and Edythe Broad Center of Regeneration Medicine and Stem Cell Research; 4Department of Neurology, University of California, San Francisco, San Francisco, CA 94143, USA; 5These authors contributed equally to this work Distinct progenitor populations generate projection neurons in an “inside-out” fashion Combinatorial transcription factor programs progressively delineate projection neuron identity I CR SCPN SVZ IMMATURE oRG VZ PROGENITORS PROGENITORS POSTMITOTIC MATURE POSTMITOTIC SP Layer IV GC Migrating II/III immature CThPN UL CPN UL CPN Tbr1 neurons DL CPN I Lhx2 Cux1/2 Astrocyte Cux1/2 Cux2 II/III IV GC Cux2 RG Rorb I IV Satb2 Couptf1 NE IP IV IV Bhlhb5 Bhlhb5 V V DL CPN I V V Lhx2 Tbr2 Emx2 Pax6 Sox6 Satb2 Ngn2 VI VI Couptf1 VI SCPN MZ Sox5 Tbr1 SP Fezf2 CP SP VI SP SP Fezf2/Ctip2 CThPN Sox5 XXXXXXXXX PP SP Tbr1 Couptf1 SVZ SVZ SVZ SVZ Fezf2/Ctip2/Otx1 SP VZ VZ VZ VZ VZ WM Subtype specicity Subtype-specic not known High expression Low expression E10.5 E11.5 E12.5 E13.5 E14.5 E15.5 P4 Expression gradients in the VZ and CP evolve into discrete domains Initially overlapping expression of controls over subtype identity that demarcate cortical areas refines as neurons differentiate E12.5 E15.5 P4 VI I CP M1M1 II/III E13.5 VZ IV Pax6 MZ P4 Emx2 Lmo4 CP V Lmo4 Bhlhb5 V Bhlhb5 See online version for legend and references. -
The Long Non-Coding RNA Gomafu Is Acutely Regulated in Response to Neuronal Activation and Involved in Schizophrenia-Associated Alternative Splicing
Molecular Psychiatry (2014) 19, 486–494 & 2014 Macmillan Publishers Limited All rights reserved 1359-4184/14 www.nature.com/mp ORIGINAL ARTICLE The long non-coding RNA Gomafu is acutely regulated in response to neuronal activation and involved in schizophrenia-associated alternative splicing G Barry1, JA Briggs2, DP Vanichkina1, EM Poth3, NJ Beveridge4,5, VS Ratnu6, SP Nayler2, K Nones7,JHu8, TW Bredy6, S Nakagawa9, F Rigo10, RJ Taft1, MJ Cairns4,5, S Blackshaw3, EJ Wolvetang2 and JS Mattick1,11,12 Schizophrenia (SZ) is a complex disease characterized by impaired neuronal functioning. Although defective alternative splicing has been linked to SZ, the molecular mechanisms responsible are unknown. Additionally, there is limited understanding of the early transcriptomic responses to neuronal activation. Here, we profile these transcriptomic responses and show that long non-coding RNAs (lncRNAs) are dynamically regulated by neuronal activation, including acute downregulation of the lncRNA Gomafu, previously implicated in brain and retinal development. Moreover, we demonstrate that Gomafu binds directly to the splicing factors QKI and SRSF1 (serine/arginine-rich splicing factor 1) and dysregulation of Gomafu leads to alternative splicing patterns that resemble those observed in SZ for the archetypal SZ-associated genes DISC1 and ERBB4. Finally, we show that Gomafu is downregulated in post-mortem cortical gray matter from the superior temporal gyrus in SZ. These results functionally link activity-regulated lncRNAs and alternative splicing in neuronal function and suggest that their dysregulation may contribute to neurological disorders. Molecular Psychiatry (2014) 19, 486–494; doi:10.1038/mp.2013.45; published online 30 April 2013 Keywords: alternative splicing; Gomafu; neuronal activation; quaking homolog; schizophrenia INTRODUCTION LncRNAs are arbitrarily defined as transcripts longer than 200 bp 10 Schizophrenia (SZ) disorder, a debilitating mental disorder that possess no protein-coding capacity. -
And Thyroid Hormone Receptor Α in Brain Cortical Layering
Correction NEUROSCIENCE Correction for “Liver X receptor β and thyroid hormone re- ceptor α in brain cortical layering,” by Xin-jie Tan, Xiao-tang Fan, Hyun-jin Kim, Ryan Butler, Paul Webb, Margaret Warner, and Jan-Åke Gustafsson, which appeared in issue 27, July 6, 2010, of Proc Natl Acad Sci USA (107:12305–12310; first pub- lished June 21, 2010; 10.1073/pnas.1006162107). The authors note that Fig. 1 appeared incorrectly. The cor- rected figure and its legend appear below. E6316–E6317 | PNAS | October 11, 2016 | vol. 113 | no. 41 www.pnas.org Downloaded by guest on October 2, 2021 CORRECTION Fig. 1. Morphological alteration of embryonic and early postnatal cortex in LXRβ−/− mice. (A–I) Sagittal sections of the E15.5 neocortex and coronal sections − − of P2 and P14 stained with cresyl violet. (A and B) At E15.5, the CP is thinner and density of neurons in the IZ is higher in LXRβ / mice (B) than in WT controls − − − − (A). (C and D) At P2, the layers II/III in LXRβ / mice (D) are thinner than in WT controls (C) and the neuronal density in layers IV and V of LXRβ / mice (D)is − − higher than that of WT controls (C). (E and F) No overall morphological difference can be observed in the cortex in LXRβ / mice (F) compared with WT controls (E) at P14. (A–F) There is no visible difference in the MZ, SVZ, and VZ at E15.5 (A and B) and layer I at P2 (C and D) or P14 (E and F) between WT and LXRβ−/− mice. -
TBR1 Regulates Autism Risk Genes in the Developing Neocortex
Downloaded from genome.cshlp.org on October 6, 2021 - Published by Cold Spring Harbor Laboratory Press Research TBR1 regulates autism risk genes in the developing neocortex James H. Notwell,1 Whitney E. Heavner,2,3 Siavash Fazel Darbandi,4 Sol Katzman,5 William L. McKenna,6 Christian F. Ortiz-Londono,6 David Tastad,6 Matthew J. Eckler,6 John L.R. Rubenstein,4 Susan K. McConnell,3 Bin Chen,6 and Gill Bejerano1,2,7 1Department of Computer Science, 2Department of Developmental Biology, 3Department of Biology, Stanford University, Stanford, California 94305, USA; 4Department of Psychiatry, University of California, San Francisco, San Francisco, California, 94143 USA; 5Center for Biomolecular Science and Engineering, University of California, Santa Cruz, Santa Cruz, California 95064 USA; 6Department of Molecular, Cell, and Developmental Biology, University of California, Santa Cruz, Santa Cruz, California 95064, USA; 7Division of Medical Genetics, Department of Pediatrics, Stanford University, Stanford, California 94305, USA Exome sequencing studies have identified multiple genes harboring de novo loss-of-function (LoF) variants in individuals with autism spectrum disorders (ASD), including TBR1, a master regulator of cortical development. We performed ChIP- seq for TBR1 during mouse cortical neurogenesis and show that TBR1-bound regions are enriched adjacent to ASD genes. ASD genes were also enriched among genes that are differentially expressed in Tbr1 knockouts, which together with the ChIP-seq data, suggests direct transcriptional regulation. Of the nine ASD genes examined, seven were misexpressed in the cortices of Tbr1 knockout mice, including six with increased expression in the deep cortical layers. ASD genes with adjacent cortical TBR1 ChIP-seq peaks also showed unusually low levels of LoF mutations in a reference human population and among Icelanders. -
Tbr1andfezf2regulate Alternate Corticofugal Neuronal Identities
The Journal of Neuroscience, January 12, 2011 • 31(2):549–564 • 549 Development/Plasticity/Repair Tbr1 and Fezf2 Regulate Alternate Corticofugal Neuronal Identities during Neocortical Development William L. McKenna,1 Jennifer Betancourt,1 Kathryn A. Larkin,1 Benjamin Abrams,1 Chao Guo,1 John L. R. Rubenstein,2 and Bin Chen1 1Department of Molecular, Cell, and Developmental Biology, University of California at Santa Cruz, Santa Cruz, California 95064, and 2Nina Ireland Laboratory of Developmental Neurobiology, Department of Psychiatry, University of California at San Francisco, San Francisco, California 94158 The molecular mechanisms regulating fate divergence of closely related, but distinct, layer 6 corticothalamic and layer 5 subcerebral projection neurons are largely unknown. We present evidence for central transcriptional mechanisms that regulate fate specification of corticothalamic (layer 6) and subcerebral (layer 5) projection neurons. We found that TBR1 promotes the identity of corticothalamic neurons and represses subcerebral fates through reducing expression of Fezf2 and CTIP2. These conclusions are based on the following: (1) In Tbr1 Ϫ/Ϫ mice, the number of cells expressing layer 6 markers was reduced, and the number of cells expressing layer 5 markers was increased. Early-born (birthdated on E11.5) neurons ectopically expressed subcerebral neuronal markers, and extended their axons into subcerebral targets. (2) Ectopic Tbr1 expression in layer 5 neurons prevented them from extending axons into the brainstem and the spinal cord. (3) Chromatin immunoprecipitation analysis using TBR1 antibodies showed that TBR1 bound to a conserved region in the Fezf2 gene. (4) Analysis of Fezf2 mutants and Tbr1 Ϫ/Ϫ; Fezf2 Ϫ/Ϫ compound mutants provided evidence that Fezf2 blocks corticotha- lamic fate in layer 5 by reducing Tbr1 expression in subcerebral neurons.