Estrogen and Serotonin – Old Dogs, New Tricks, Implications for Pancreatic Beta-Cell Function
Total Page:16
File Type:pdf, Size:1020Kb
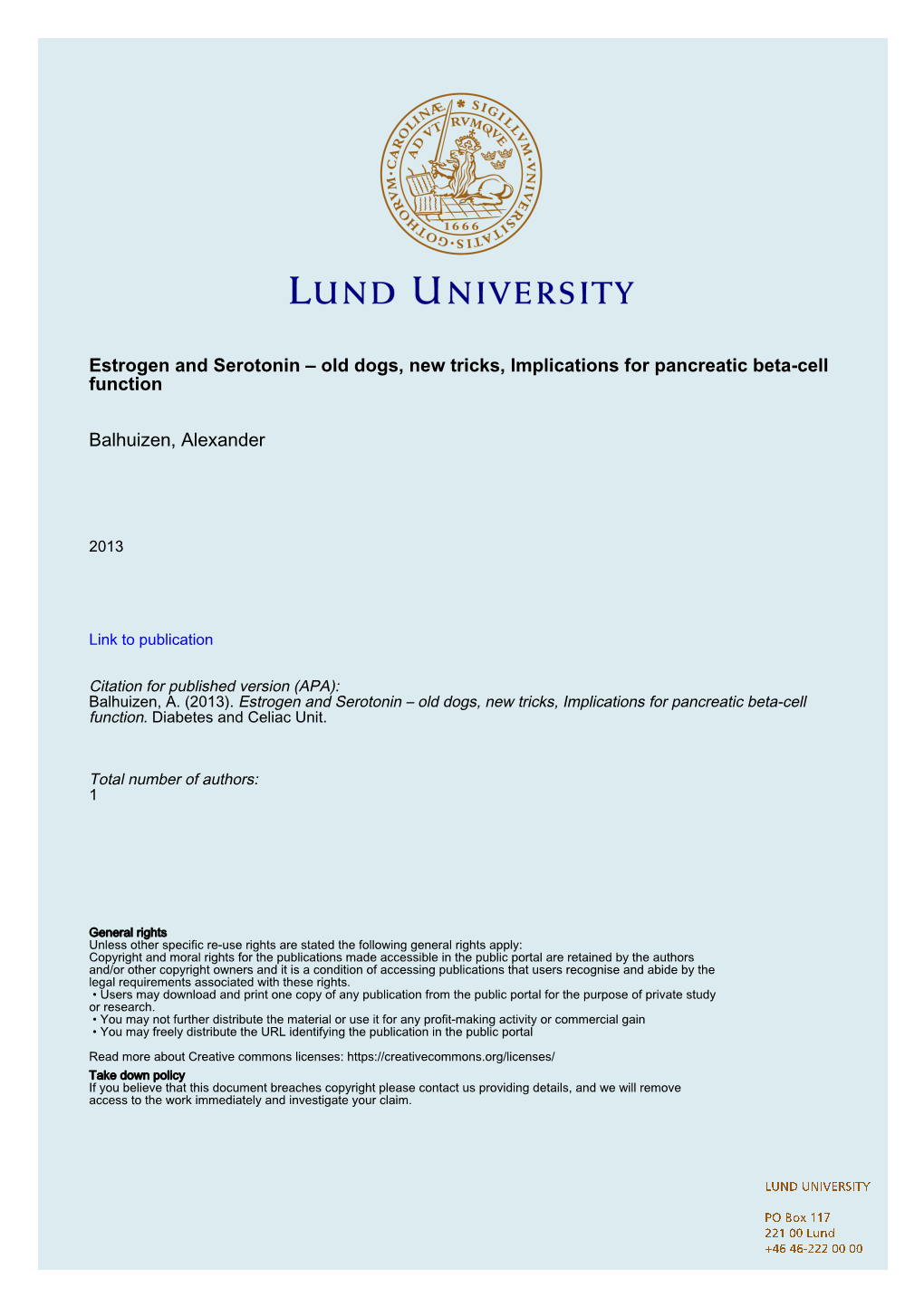
Load more
Recommended publications
-
Targeting Lysophosphatidic Acid in Cancer: the Issues in Moving from Bench to Bedside
View metadata, citation and similar papers at core.ac.uk brought to you by CORE provided by IUPUIScholarWorks cancers Review Targeting Lysophosphatidic Acid in Cancer: The Issues in Moving from Bench to Bedside Yan Xu Department of Obstetrics and Gynecology, Indiana University School of Medicine, 950 W. Walnut Street R2-E380, Indianapolis, IN 46202, USA; [email protected]; Tel.: +1-317-274-3972 Received: 28 August 2019; Accepted: 8 October 2019; Published: 10 October 2019 Abstract: Since the clear demonstration of lysophosphatidic acid (LPA)’s pathological roles in cancer in the mid-1990s, more than 1000 papers relating LPA to various types of cancer were published. Through these studies, LPA was established as a target for cancer. Although LPA-related inhibitors entered clinical trials for fibrosis, the concept of targeting LPA is yet to be moved to clinical cancer treatment. The major challenges that we are facing in moving LPA application from bench to bedside include the intrinsic and complicated metabolic, functional, and signaling properties of LPA, as well as technical issues, which are discussed in this review. Potential strategies and perspectives to improve the translational progress are suggested. Despite these challenges, we are optimistic that LPA blockage, particularly in combination with other agents, is on the horizon to be incorporated into clinical applications. Keywords: Autotaxin (ATX); ovarian cancer (OC); cancer stem cell (CSC); electrospray ionization tandem mass spectrometry (ESI-MS/MS); G-protein coupled receptor (GPCR); lipid phosphate phosphatase enzymes (LPPs); lysophosphatidic acid (LPA); phospholipase A2 enzymes (PLA2s); nuclear receptor peroxisome proliferator-activated receptor (PPAR); sphingosine-1 phosphate (S1P) 1. -
Glucocorticoid Regulation of the G-Protein Coupled Estrogen Receptor (GPER) in Mouse Hippocampal Neurons
Glucocorticoid Regulation of the G-Protein Coupled Estrogen Receptor (GPER) in Mouse Hippocampal Neurons by Kate Colleen Eliza Nicholson A Thesis presented to The University of Guelph In partial fulfilment of requirements for the degree of Master of Science in Biomedical Sciences Guelph, Ontario, Canada © Kate Colleen Eliza Nicholson, August, 2019 ABSTRACT GLUCOCORTICOID REGULATION OF THE G-PROTEIN COUPLED ESTROGEN RECEPTOR (GPER) IN MOUSE HIPPOCAMPAL NEURONS Kate Colleen Eliza Nicholson Advisor: University of Guelph, 2019 Dr. Neil J. MacLusky The most prevalent estrogen, 17β-estradiol, binds the non-classical G-protein coupled estrogen receptor (GPER) with high affinity resulting in rapid activation of the c- jun N terminal kinase (JNK) pathway. GPER activation mediates some of the rapid neurotrophic and memory-enhancing effects of 17β-estradiol in the female hippocampus. However, exposure to stressful stimuli may impair these beneficial effects. This thesis characterizes neurosteroid receptor expression in murine-derived mHippoE cell lines that are subsequently used to investigate the glucocorticoid regulation of GPER protein expression and functional activation. This thesis demonstrates that 24-hour treatment with a glucocorticoid receptor agonist reduces GPER protein expression and activation of JNK in female-derived mHippoE-14s. Using an in vivo model, treatment with glucocorticoids significantly reduces hippocampal activation of JNK in female ovariectomized CD1 mice. Collectively, this thesis uses in vitro and in vivo models to characterize glucocorticoid regulation of GPER expression and signalling in female murine hippocampal neurons. ACKNOWLEDGEMENTS To Dr. MacLusky: I would like to thank you for inspiring my passion for science and pursuit of knowledge. Over the past 2 years, you have provided me with countless opportunities to grow as a young researcher and I am tremendously grateful for this. -
G Protein-Coupled Estrogen Receptor Inhibits the P2Y Receptor-Mediated Ca2+ Signaling Pathway in Human Airway Epithelia
Pflugers Arch - Eur J Physiol DOI 10.1007/s00424-016-1840-7 SIGNALING AND CELL PHYSIOLOGY G protein-coupled estrogen receptor inhibits the P2Y receptor-mediated Ca2+ signaling pathway in human airway epithelia Yuan Hao1 & Alison W. Chow1 & Wallace C. Yip 1 & Chi H. Li1 & Tai F. Wan 1 & Benjamin C. Tong2 & King H. Cheung2 & Wood Y. Chan 1 & Yangchao Chen 1 & Christopher H. Cheng1 & Wing H. Ko1 Received: 21 January 2016 /Revised: 11 May 2016 /Accepted: 22 May 2016 # The Author(s) 2016. This article is published with open access at Springerlink.com Abstract P2Y receptor activation causes the release of in- inhibitory effects of G1 or E2 on P2Y receptor-mediated flammatory cytokines in the bronchial epithelium, whereas Ca2+ mobilization and cytokine secretion were due to G protein-coupled estrogen receptor (GPER), a novel estrogen GPER-mediated activation of a cAMP-dependent PKA path- (E2) receptor, may play an anti-inflammatory role in this pro- way. This study has reported, for the first time, the expression cess. We investigated the cellular mechanisms underlying the and function of GPER as an anti-inflammatory component in inhibitory effect of GPER activation on the P2Y receptor- human bronchial epithelia, which may mediate through its mediated Ca2+ signaling pathway and cytokine production in opposing effects on the pro‐inflammatory pathway activated airway epithelia. Expression of GPER in primary human by the P2Y receptors in inflamed airway epithelia. bronchial epithelial (HBE) or 16HBE14o- cells was con- firmed on both the mRNA and protein levels. Stimulation of Keywords GPER . P2Y receptor signaling pathway . Human HBE or 16HBE14o- cells with E2 or G1, a specific agonist of . -
November Packet
L Buckman Dire cl Diversion Date: October 22,2018 To: Buckman Direct Diversion Board From: Michael Dozier, BDD Operations Superintendent .AAD Subject: Update on BDD Operations for the Month of October 2018 ITEM: 1. This memorandum is to update the Buckman Direct Diversion Board (BDDB) on BDD operations during the month of October 2018. The BDD diversions and deliveries have averaged, in Million Gallons Per Day (MOD) as follows: a. Raw water diversions: 5.66 MOD b. Drinking water deliveries through Booster Station 4N5A: 5.08 MOD c. Raw water delivery to Las Campanas at BS2A: 0.53 MOD d. Onsite treated and non-treated water storage: 0.05 MOD Average 2. The BDD is providing approximately 81% percent of the water supply to the City and County for the month. 3. The BDD year-to-date diversions are depicted below: Year-To-Date Comparison 350.00 , I ®.' Buckman Direct Diversion • 341 Caja del Rio Rd. • Santa Fe, NM 87506 1 4. Background Diversion tables: Buckman Direct Diversion Monthly SJC and Native Diversions Oct-18 In Acre-Feet Total SD-03418 SP-4842 SP-2847-E SP-2847-N-A All Partners SJC+ RGNative Month RG Native SJCCall SJCCall Conveyance Native LAS SJCCall COUNTY CITY LASCAMPANAS Losses Rights CAMPANAS Total JAN 380.137 77.791 0.000 302.346 302.346 0.000 3.023 FEB 336.287 66.413 0.000 269.874 169.874 0.000 2.699 MAR 362.730 266.898 0.000 95.832 95.832 0.000 0.958 APR 661.333 568.669 0.000 92.664 92.664 0.000 0.927 MAY 933.072 340.260 0.000 592.812 481.647 111.165 5.928 JUN 873.384 44.160 0.000 829.224 693.960 135.264 8.292 JUL 807.939 -
Anti-Obesity Therapy: from Rainbow Pills to Polyagonists
1521-0081/70/4/712–746$35.00 https://doi.org/10.1124/pr.117.014803 PHARMACOLOGICAL REVIEWS Pharmacol Rev 70:712–746, October 2018 Copyright © 2018 The Author(s). This is an open access article distributed under the CC BY Attribution 4.0 International license. ASSOCIATE EDITOR: BIRGITTE HOLST Anti-Obesity Therapy: from Rainbow Pills to Polyagonists T. D. Müller, C. Clemmensen, B. Finan, R. D. DiMarchi, and M. H. Tschöp Institute for Diabetes and Obesity, Helmholtz Diabetes Center, Helmholtz Zentrum München, German Research Center for Environmental Health, Neuherberg, Germany (T.D.M., C.C., M.H.T.); German Center for Diabetes Research, Neuherberg, Germany (T.D.M., C.C., M.H.T.); Department of Chemistry, Indiana University, Bloomington, Indiana (B.F., R.D.D.); and Division of Metabolic Diseases, Technische Universität München, Munich, Germany (M.H.T.) Abstract ....................................................................................713 I. Introduction . ..............................................................................713 II. Bariatric Surgery: A Benchmark for Efficacy ................................................714 III. The Chronology of Modern Weight-Loss Pharmacology . .....................................715 A. Thyroid Hormones ......................................................................716 B. 2,4-Dinitrophenol .......................................................................716 C. Amphetamines. ........................................................................717 Downloaded from 1. Methamphetamine -
G Protein-Coupled Receptors Function As Cell Membrane Receptors for the Steroid Hormone 20-Hydroxyecdysone Xiao-Fan Zhao
Zhao Cell Communication and Signaling (2020) 18:146 https://doi.org/10.1186/s12964-020-00620-y REVIEW Open Access G protein-coupled receptors function as cell membrane receptors for the steroid hormone 20-hydroxyecdysone Xiao-Fan Zhao Abstract G protein-coupled receptors (GPCRs) are cell membrane receptors for various ligands. Recent studies have suggested that GPCRs transmit animal steroid hormone signals. Certain GPCRs have been shown to bind steroid hormones, for example, G protein-coupled estrogen receptor 1 (GPER1) binds estrogen in humans, and Drosophila dopamine/ecdysteroid receptor (DopEcR) binds the molting hormone 20-hydroxyecdysone (20E) in insects. This review summarizes the research progress on GPCRs as animal steroid hormone cell membrane receptors, including the nuclear and cell membrane receptors of steroid hormones in mammals and insects, the 20E signaling cascade via GPCRs, termination of 20E signaling, and the relationship between genomic action and the nongenomic action of 20E. Studies indicate that 20E induces a signal via GPCRs to regulate rapid cellular responses, including rapid Ca2+ release from the endoplasmic reticulum and influx from the extracellular medium, as well as rapid protein phosphorylation and subcellular translocation. 20E via the GPCR/Ca2+/PKC/signaling axis and the GPCR/cAMP/PKA- signaling axis regulates gene transcription by adjusting transcription complex formation and DNA binding activity. GPCRs can bind 20E in the cell membrane and after being isolated, suggesting GPCRs as cell membrane receptors of 20E. This review deepens our understanding of GPCRs as steroid hormone cell membrane receptors and the GPCR-mediated signaling pathway of 20E (20E-GPCR pathway), which will promote further study of steroid hormone signaling via GPCRs, and presents GPCRs as targets to explore new pharmaceutical materials to treat steroid hormone-related diseases or control pest insects. -
Download (PDF)
Supplemental Information Biological and Pharmaceutical Bulletin Promoter Methylation Profiles between Human Lung Adenocarcinoma Multidrug Resistant A549/Cisplatin (A549/DDP) Cells and Its Progenitor A549 Cells Ruiling Guo, Guoming Wu, Haidong Li, Pin Qian, Juan Han, Feng Pan, Wenbi Li, Jin Li, and Fuyun Ji © 2013 The Pharmaceutical Society of Japan Table S1. Gene categories involved in biological functions with hypomethylated promoter identified by MeDIP-ChIP analysis in lung adenocarcinoma MDR A549/DDP cells compared with its progenitor A549 cells Different biological Genes functions transcription factor MYOD1, CDX2, HMX1, THRB, ARNT2, ZNF639, HOXD13, RORA, FOXO3, HOXD10, CITED1, GATA1, activity HOXC6, ZGPAT, HOXC8, ATOH1, FLI1, GATA5, HOXC4, HOXC5, PHTF1, RARB, MYST2, RARG, SIX3, FOXN1, ZHX3, HMG20A, SIX4, NR0B1, SIX6, TRERF1, DDIT3, ASCL1, MSX1, HIF1A, BAZ1B, MLLT10, FOXG1, DPRX, SHOX, ST18, CCRN4L, TFE3, ZNF131, SOX5, TFEB, MYEF2, VENTX, MYBL2, SOX8, ARNT, VDR, DBX2, FOXQ1, MEIS3, HOXA6, LHX2, NKX2-1, TFDP3, LHX6, EWSR1, KLF5, SMAD7, MAFB, SMAD5, NEUROG1, NR4A1, NEUROG3, GSC2, EN2, ESX1, SMAD1, KLF15, ZSCAN1, VAV1, GAS7, USF2, MSL3, SHOX2, DLX2, ZNF215, HOXB2, LASS3, HOXB5, ETS2, LASS2, DLX5, TCF12, BACH2, ZNF18, TBX21, E2F8, PRRX1, ZNF154, CTCF, PAX3, PRRX2, CBFA2T2, FEV, FOS, BARX1, PCGF2, SOX15, NFIL3, RBPJL, FOSL1, ALX1, EGR3, SOX14, FOXJ1, ZNF92, OTX1, ESR1, ZNF142, FOSB, MIXL1, PURA, ZFP37, ZBTB25, ZNF135, HOXC13, KCNH8, ZNF483, IRX4, ZNF367, NFIX, NFYB, ZBTB16, TCF7L1, HIC1, TSC22D1, TSC22D2, REXO4, POU3F2, MYOG, NFATC2, ENO1, -
Sex Steroids Regulate Skin Pigmentation Through Nonclassical
RESEARCH ARTICLE Sex steroids regulate skin pigmentation through nonclassical membrane-bound receptors Christopher A Natale1, Elizabeth K Duperret1, Junqian Zhang1, Rochelle Sadeghi1, Ankit Dahal1, Kevin Tyler O’Brien2, Rosa Cookson2, Jeffrey D Winkler2, Todd W Ridky1* 1Department of Dermatology, Perelman School of Medicine, University of Pennsylvania, Philadelphia, United States; 2Department of Chemistry, University of Pennsylvania, Philadelphia, United States Abstract The association between pregnancy and altered cutaneous pigmentation has been documented for over two millennia, suggesting that sex hormones play a role in regulating epidermal melanocyte (MC) homeostasis. Here we show that physiologic estrogen (17b-estradiol) and progesterone reciprocally regulate melanin synthesis. This is intriguing given that we also show that normal primary human MCs lack classical estrogen or progesterone receptors (ER or PR). Utilizing both genetic and pharmacologic approaches, we establish that sex steroid effects on human pigment synthesis are mediated by the membrane-bound, steroid hormone receptors G protein-coupled estrogen receptor (GPER), and progestin and adipoQ receptor 7 (PAQR7). Activity of these receptors was activated or inhibited by synthetic estrogen or progesterone analogs that do not bind to ER or PR. As safe and effective treatment options for skin pigmentation disorders are limited, these specific GPER and PAQR7 ligands may represent a novel class of therapeutics. DOI: 10.7554/eLife.15104.001 *For correspondence: ridky@mail. -
Downloaded from Bioscientifica.Com at 09/25/2021 05:16:42PM Via Free Access
28 2 Endocrine-Related E B Blatt et al. Overcoming oncogene 28:2 R31–R46 Cancer addiction in BCa and PCa REVIEW Overcoming oncogene addiction in breast and prostate cancers: a comparative mechanistic overview Eliot B Blatt1, Noa Kopplin1, Shourya Kumar1, Ping Mu2, Suzanne D Conzen3 and Ganesh V Raj1,4 1Department of Urology, University of Texas Southwestern Medical Center, Dallas, Texas, USA 2Department of Molecular Biology, University of Texas Southwestern Medical Center, Dallas, Texas, USA 3Department of Internal Medicine, University of Texas Southwestern Medical Center, Dallas, Texas, USA 4Department of Pharmacology, University of Texas Southwestern Medical Center, Dallas, Texas, USA Correspondence should be addressed to G V Raj: [email protected] Abstract Prostate cancer (PCa) and breast cancer (BCa) are both hormone-dependent cancers Key Words that require the androgen receptor (AR) and estrogen receptor (ER, ESR1) for growth and f endocrine therapy proliferation, respectively. Endocrine therapies that target these nuclear receptors (NRs) resistance provide significant clinical benefit for metastatic patients. However, these therapeutic f androgen receptor strategies are seldom curative and therapy resistance is prevalent. Because the vast f estrogen receptor majority of therapy-resistant PCa and BCa remain dependent on the augmented activity f oncogene of their primary NR driver, common mechanisms of resistance involve enhanced NR signaling through overexpression, mutation, or alternative splicing of the receptor, coregulator alterations, and increased intracrine hormonal synthesis. In addition, a significant subset of endocrine therapy-resistant tumors become independent of their primary NR and switch to alternative NR or transcriptional drivers. While these hormone-dependent cancers generally employ similar mechanisms of endocrine therapy resistance, distinct differences between the two tumor types have been observed. -
I GPER/GPR30 ESTROGEN RECEPTOR
GPER/GPR30 ESTROGEN RECEPTOR: A TARGET FOR PAIN MODULATION A Dissertation Submitted to The Temple University Graduate Board In Partial Fulfillment of the Requirements for the Degree of Doctor of Philosophy By Elena Deliu May, 2012 Examination Committee Members: Dr. Nae J Dun (Advisor), Dept. of Pharmacology, Temple University Dr. Mary E Abood, Dept. of Anatomy and Cell Biology, Temple University Dr. Barrie Ashby, Dept. of Pharmacology, Temple University Dr. Eugen Brailoiu, Dept. of Pharmacology, Temple University Dr. Ellen M Unterwald, Dept. of Pharmacology, Temple University Dr. Hreday Sapru, (External Examiner), Depts. of Neurosciences, Neurosurgery & Pharmacology/Physiology, UMDNJ-NJMS i © 2012 By Elena Deliu All Rights Reserved ii ABSTRACT GPER/GPR30 ESTROGEN RECEPTOR: A TARGET FOR PAIN MODULATION Elena Deliu Doctor of Philosophy Temple University, 2012 Doctoral Advisory Committee Chair: Nae J. Dun, Ph.D. The G protein-coupled estrogen receptor GPER/GPER1, also known as GPR30, was originally cloned as an orphan receptor and later shown to be specifically activated by 17- β-estradiol. This has led to its classification as an estrogen receptor and expanded the perspective on the mechanisms underlying the rapid estrogenic effects reported over the years. GPER is strongly expressed in the central nervous system and peripheral tissues and appears to be involved in a wide variety of physiological and pathological processes. Estrogens are known to alter the processing of nociceptive sensory information and analgesic responses in the central nervous system. Both analgesic and pro-nociceptive effects of estrogens have been reported. Some pro-algesic estrogenic responses have a short latency, suggesting a non-genomic mechanism of action. -
Binding of Androgen- and Estrogen-Like Flavonoids to Their Cognate (Non)Nuclear Receptors: a Comparison by Computational Prediction
molecules Article Binding of Androgen- and Estrogen-Like Flavonoids to Their Cognate (Non)Nuclear Receptors: A Comparison by Computational Prediction Giulia D’Arrigo 1, Eleonora Gianquinto 1, Giulia Rossetti 2,3,4 , Gabriele Cruciani 5, Stefano Lorenzetti 6,* and Francesca Spyrakis 1,* 1 Department of Drug Science and Technology, University of Turin, Via Giuria 9, 10125 Turin, Italy; [email protected] (G.D.); [email protected] (E.G.) 2 Institute for Neuroscience and Medicine (INM-9) and Institute for Advanced Simulations (IAS-5) “Computational Biomedicine”, Forschungszentrum Jülich, 52425 Jülich, Germany 3 Jülich Supercomputing Center (JSC), Forschungszentrum Jülich, 52425 Jülich, Germany 4 Department of Neurology, RWTH, Aachen University, 52074 Aachen, Germany; [email protected] 5 Department of Chemistry, Biology and Biotechnology, University of Perugia, 06123 Perugia, Italy; [email protected] 6 Istituto Superiore di Sanità (ISS), Department of Food Safety, Nutrition and Veterinary Public Health, Viale Regina Elena 299, 00161 Rome, Italy * Correspondence: [email protected] (S.L.); [email protected] (F.S.) Abstract: Flavonoids are plant bioactives that are recognized as hormone-like polyphenols because of Citation: D’Arrigo, G.; Gianquinto, their similarity to the endogenous sex steroids 17β-estradiol and testosterone, and to their estrogen- E.; Rossetti, G.; Cruciani, G.; and androgen-like activity. Most efforts to verify flavonoid binding to nuclear receptors (NRs) and Lorenzetti, S.; Spyrakis, F. Binding of explain their action have been focused on ERα, while less attention has been paid to other nuclear Androgen- and Estrogen-Like and non-nuclear membrane androgen and estrogen receptors. Here, we investigate six flavonoids Flavonoids to Their Cognate (apigenin, genistein, luteolin, naringenin, quercetin, and resveratrol) that are widely present in fruits (Non)Nuclear Receptors: A and vegetables, and often used as replacement therapy in menopause. -
Maternal Testosterone Exposure Increases Anxiety-Like Behavior and Impacts the Limbic System in the Offspring
Maternal testosterone exposure increases anxiety-like behavior and impacts the limbic system in the offspring Min Hua,b,1, Jennifer Elise Richardc,1, Manuel Maliqueoa,d,1, Milana Kokosarc, Romina Fornesa, Anna Benrickc, Thomas Janssone, Claes Ohlssonf, Xiaoke Wub,2, Karolina Patrycja Skibickac,3, and Elisabet Stener-Victorina,2,3 aDepartment of Physiology and Pharmacology, Karolinska Institutet, 17177 Stockholm, Sweden; bDepartment of Obstetrics and Gynecology, First Affiliated Hospital, Heilongjiang University of Chinese Medicine, 150040 Harbin, China; cDepartment of Physiology, Institute of Neuroscience and Physiology, Sahlgrenska Academy, University of Gothenburg, 405 30 Gothenburg, Sweden; dEndocrinology and Metabolism Laboratory, Department of Medicine West Division, School of Medicine, University of Chile, 8320000 Santiago, Chile; eDepartment of Obstetrics & Gynecology, University of Colorado Anschutz Medical Campus, Aurora, CO 80045; and fCentre for Bone and Arthritis Research, Department of Internal Medicine and Clinical Nutrition, Institute of Medicine, Sahlgrenska Academy, University of Gothenburg, 413 45 Gothenburg, Sweden Edited by Bruce S. McEwen, The Rockefeller University, New York, NY, and approved October 1, 2015 (received for review April 30, 2015) During pregnancy, women with polycystic ovary syndrome (PCOS) Maternal testosterone levels in humans have been shown to af- display high circulating androgen levels that may affect the fetus fect brain morphology and function (6) and to be correlated to and increase the risk of mood disorders in offspring. This study neural development and mental function (7). There is evidence for investigated whether maternal androgen excess causes anxiety-like a crucial role of the hippocampus and the amygdala in the devel- behavior in offspring mimicking anxiety disorders in PCOS.