Detection of Baryonic Acoustic Oscillations in the Matter Power Spectrum
Total Page:16
File Type:pdf, Size:1020Kb
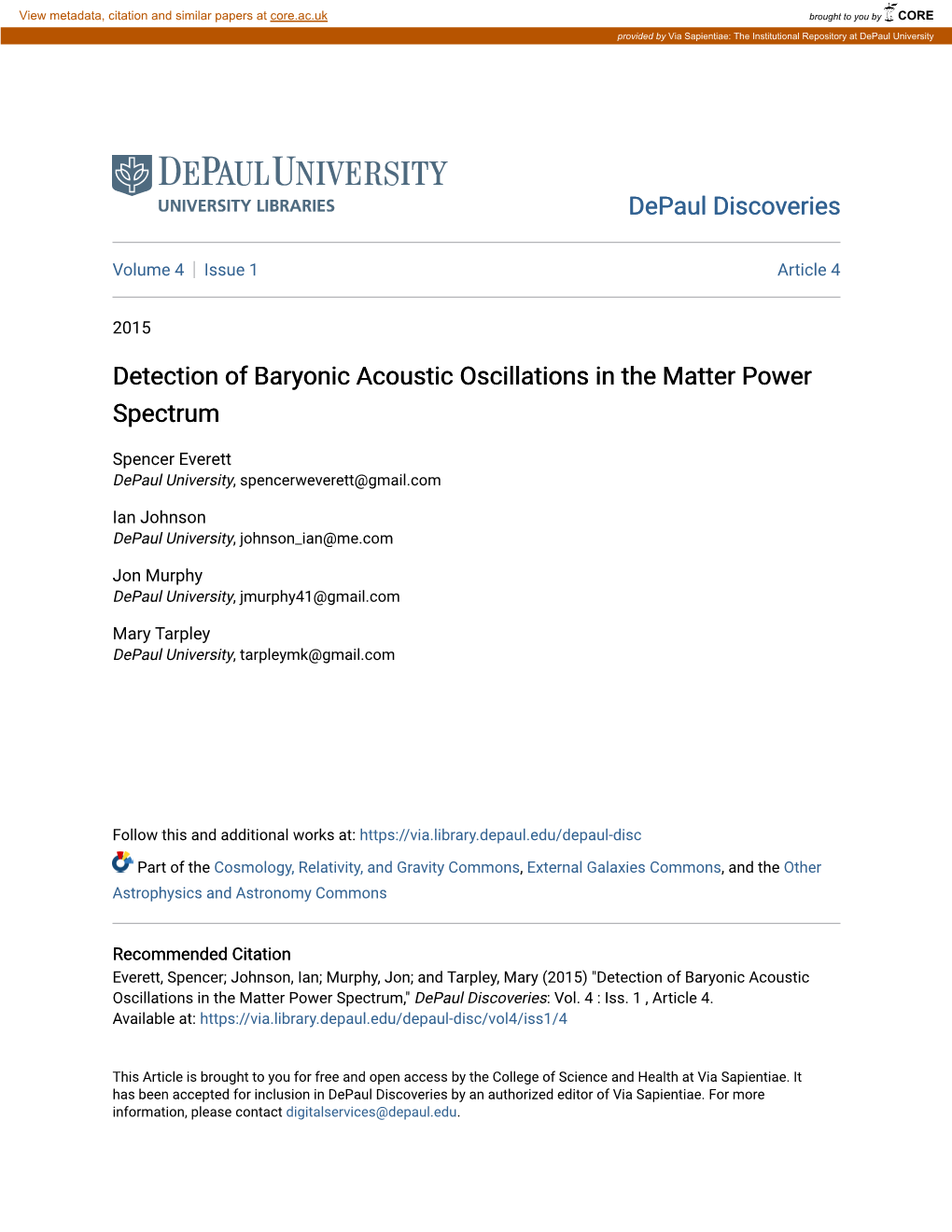
Load more
Recommended publications
-
String Theory and Pre-Big Bang Cosmology
IL NUOVO CIMENTO 38 C (2015) 160 DOI 10.1393/ncc/i2015-15160-8 Colloquia: VILASIFEST String theory and pre-big bang cosmology M. Gasperini(1)andG. Veneziano(2) (1) Dipartimento di Fisica, Universit`a di Bari - Via G. Amendola 173, 70126 Bari, Italy and INFN, Sezione di Bari - Bari, Italy (2) CERN, Theory Unit, Physics Department - CH-1211 Geneva 23, Switzerland and Coll`ege de France - 11 Place M. Berthelot, 75005 Paris, France received 11 January 2016 Summary. — In string theory, the traditional picture of a Universe that emerges from the inflation of a very small and highly curved space-time patch is a possibility, not a necessity: quite different initial conditions are possible, and not necessarily unlikely. In particular, the duality symmetries of string theory suggest scenarios in which the Universe starts inflating from an initial state characterized by very small curvature and interactions. Such a state, being gravitationally unstable, will evolve towards higher curvature and coupling, until string-size effects and loop corrections make the Universe “bounce” into a standard, decreasing-curvature regime. In such a context, the hot big bang of conventional cosmology is replaced by a “hot big bounce” in which the bouncing and heating mechanisms originate from the quan- tum production of particles in the high-curvature, large-coupling pre-bounce phase. Here we briefly summarize the main features of this inflationary scenario, proposed a quarter century ago. In its simplest version (where it represents an alternative and not a complement to standard slow-roll inflation) it can produce a viable spectrum of density perturbations, together with a tensor component characterized by a “blue” spectral index with a peak in the GHz frequency range. -
Big Bang Blunder Bursts the Multiverse Bubble
WORLD VIEW A personal take on events IER P P. PA P. Big Bang blunder bursts the multiverse bubble Premature hype over gravitational waves highlights gaping holes in models for the origins and evolution of the Universe, argues Paul Steinhardt. hen a team of cosmologists announced at a press world will be paying close attention. This time, acceptance will require conference in March that they had detected gravitational measurements over a range of frequencies to discriminate from fore- waves generated in the first instants after the Big Bang, the ground effects, as well as tests to rule out other sources of confusion. And Worigins of the Universe were once again major news. The reported this time, the announcements should be made after submission to jour- discovery created a worldwide sensation in the scientific community, nals and vetting by expert referees. If there must be a press conference, the media and the public at large (see Nature 507, 281–283; 2014). hopefully the scientific community and the media will demand that it According to the team at the BICEP2 South Pole telescope, the is accompanied by a complete set of documents, including details of the detection is at the 5–7 sigma level, so there is less than one chance systematic analysis and sufficient data to enable objective verification. in two million of it being a random occurrence. The results were The BICEP2 incident has also revealed a truth about inflationary the- hailed as proof of the Big Bang inflationary theory and its progeny, ory. The common view is that it is a highly predictive theory. -
DARK AGES of the Universe the DARK AGES of the Universe Astronomers Are Trying to fill in the Blank Pages in Our Photo Album of the Infant Universe by Abraham Loeb
THE DARK AGES of the Universe THE DARK AGES of the Universe Astronomers are trying to fill in the blank pages in our photo album of the infant universe By Abraham Loeb W hen I look up into the sky at night, I often wonder whether we humans are too preoccupied with ourselves. There is much more to the universe than meets the eye on earth. As an astrophysicist I have the privilege of being paid to think about it, and it puts things in perspective for me. There are things that I would otherwise be bothered by—my own death, for example. Everyone will die sometime, but when I see the universe as a whole, it gives me a sense of longevity. I do not care so much about myself as I would otherwise, because of the big picture. Cosmologists are addressing some of the fundamental questions that people attempted to resolve over the centuries through philosophical thinking, but we are doing so based on systematic observation and a quantitative methodology. Perhaps the greatest triumph of the past century has been a model of the uni- verse that is supported by a large body of data. The value of such a model to our society is sometimes underappreciated. When I open the daily newspaper as part of my morning routine, I often see lengthy de- scriptions of conflicts between people about borders, possessions or liberties. Today’s news is often forgotten a few days later. But when one opens ancient texts that have appealed to a broad audience over a longer period of time, such as the Bible, what does one often find in the opening chap- ter? A discussion of how the constituents of the universe—light, stars, life—were created. -
Origin and Evolution of the Universe Baryogenesis
Physics 224 Spring 2008 Origin and Evolution of the Universe Baryogenesis Lecture 18 - Monday Mar 17 Joel Primack University of California, Santa Cruz Post-Inflation Baryogenesis: generation of excess of baryon (and lepton) number compared to anti-baryon (and anti-lepton) number. in order to create the observed baryon number today it is only necessary to create an excess of about 1 quark and lepton for every ~109 quarks+antiquarks and leptons +antileptons. Other things that might happen Post-Inflation: Breaking of Pecci-Quinn symmetry so that the observable universe is composed of many PQ domains. Formation of cosmic topological defects if their amplitude is small enough not to violate cosmological bounds. There is good evidence that there are no large regions of antimatter (Cohen, De Rujula, and Glashow, 1998). It was Andrei Sakharov (1967) who first suggested that the baryon density might not represent some sort of initial condition, but might be understandable in terms of microphysical laws. He listed three ingredients to such an understanding: 1. Baryon number violation must occur in the fundamental laws. At very early times, if baryon number violating interactions were in equilibrium, then the universe can be said to have “started” with zero baryon number. Starting with zero baryon number, baryon number violating interactions are obviously necessary if the universe is to end up with a non-zero asymmetry. As we will see, apart from the philosophical appeal of these ideas, the success of inflationary theory suggests that, shortly after the big bang, the baryon number was essentially zero. 2. CP-violation: If CP (the product of charge conjugation and parity) is conserved, every reaction which produces a particle will be accompanied by a reaction which produces its antiparticle at precisely the same rate, so no baryon number can be generated. -
The Big Bang Cosmological Model: Theory and Observations
THE BIG BANG COSMOLOGICAL MODEL: THEORY AND OBSERVATIONS MARTINA GERBINO INFN, sezione di Ferrara ISAPP 2021 Valencia July 22st, 2021 1 Structure formation Maps of CMB anisotropies show the Universe as it was at the time of recombination. The CMB field is isotropic and !" the rms fluctuations (in total intensity) are very small, < | |# > ~10$% (even smaller in polarization). Density " perturbations � ≡ ��/� are proportional to CMB fluctuations. It is possible to show that, at recombination, perturbations could be from a few (for baryons) to at most 100 times (for CDM) larger than CMB fluctuations. We need a theory of structure formation that allows to link the tiny perturbations at z~1100 to the large scale structure of the Universe we observe today (from galaxies to clusters and beyond). General picture: small density perturbations grow via gravitational instability (Jeans mechanism). The growth is suppressed during radiation-domination and eventually kicks-off after the time of equality (z~3000). When inside the horizon, perturbations grow proportional to the scale factor as long as they are in MD and remain in the linear regime (� ≪ 1). M. GERBINO 2 ISAPP-VALENCIA, 22 JULY 2021 Preliminaries & (⃗ $&) Density contrast �(�⃗) ≡ and its Fourier expansion � = ∫ �+� �(�⃗) exp(��. �⃗) &) * Credits: Kolb&Turner 2� � � ≡ ; � = ; � = ��; � ,-./ � ,-./ � � �+, �� � ≡ �+ �; � � = �(�)$+� ∝ 6 ,-./ -01 6 �+/#, �� �+ �(�) ≈ �3 -01 2� The amplitude of perturbations as they re-enter the horizon is given by the primordial power spectrum. Once perturbations re-enter the horizon, micro-physics processes modify the primordial spectrum Scale factor M. GERBINO 3 ISAPP-VALENCIA, 22 JULY 2021 Jeans mechanism (non-expanding) The Newtonian motion of a perfect fluid is decribed via the Eulerian equations. -
What Happened Before the Big Bang?
Quarks and the Cosmos ICHEP Public Lecture II Seoul, Korea 10 July 2018 Michael S. Turner Kavli Institute for Cosmological Physics University of Chicago 100 years of General Relativity 90 years of Big Bang 50 years of Hot Big Bang 40 years of Quarks & Cosmos deep connections between the very big & the very small 100 years of QM & atoms 50 years of the “Standard Model” The Universe is very big (billions and billions of everything) and often beyond the reach of our minds and instruments Big ideas and powerful instruments have enabled revolutionary progress a very big idea connections between quarks & the cosmos big telescopes on the ground Hawaii Chile and in space: Hubble, Spitzer, Chandra, and Fermi at the South Pole basics of our Universe • 100 billion galaxies • each lit with the light of 100 billion stars • carried away from each other by expanding space from a • big bang beginning 14 billion yrs ago Hubble (1925): nebulae are “island Universes” Universe comprised of billions of galaxies Hubble Deep Field: one ten millionth of the sky, 10,000 galaxies 100 billion galaxies in the observable Universe Universe is expanding and had a beginning … Hubble, 1929 Signature of big bang beginning Einstein: Big Bang = explosion of space with galaxies carried along The big questions circa 1978 just two numbers: H0 and q0 Allan Sandage, Hubble’s “student” H0: expansion rate (slope age) q0: deceleration (“droopiness” destiny) … tens of astronomers working (alone) to figure it all out Microwave echo of the big bang Hot MichaelBig S Turner Bang -
Cosmic Microwave Background
1 29. Cosmic Microwave Background 29. Cosmic Microwave Background Revised August 2019 by D. Scott (U. of British Columbia) and G.F. Smoot (HKUST; Paris U.; UC Berkeley; LBNL). 29.1 Introduction The energy content in electromagnetic radiation from beyond our Galaxy is dominated by the cosmic microwave background (CMB), discovered in 1965 [1]. The spectrum of the CMB is well described by a blackbody function with T = 2.7255 K. This spectral form is a main supporting pillar of the hot Big Bang model for the Universe. The lack of any observed deviations from a 7 blackbody spectrum constrains physical processes over cosmic history at redshifts z ∼< 10 (see earlier versions of this review). Currently the key CMB observable is the angular variation in temperature (or intensity) corre- lations, and to a growing extent polarization [2–4]. Since the first detection of these anisotropies by the Cosmic Background Explorer (COBE) satellite [5], there has been intense activity to map the sky at increasing levels of sensitivity and angular resolution by ground-based and balloon-borne measurements. These were joined in 2003 by the first results from NASA’s Wilkinson Microwave Anisotropy Probe (WMAP)[6], which were improved upon by analyses of data added every 2 years, culminating in the 9-year results [7]. In 2013 we had the first results [8] from the third generation CMB satellite, ESA’s Planck mission [9,10], which were enhanced by results from the 2015 Planck data release [11, 12], and then the final 2018 Planck data release [13, 14]. Additionally, CMB an- isotropies have been extended to smaller angular scales by ground-based experiments, particularly the Atacama Cosmology Telescope (ACT) [15] and the South Pole Telescope (SPT) [16]. -
Banks-Zaks Cosmology, Inflation, and the Big Bang Singularity
Prepared for submission to JCAP Banks-Zaks Cosmology, Inflation, and the Big Bang Singularity Michal Artymowski, Ido Ben-Dayan, Utkarsh Kumar Physics Department, Ariel University, Ariel 40700, Israel E-mail: [email protected], [email protected], [email protected] Abstract. We consider the thermodynamical behavior of Banks-Zaks theory close to the conformal point in a cosmological setting. Due to the anomalous dimension, the resulting pressure and energy density deviate from that of radiation and result in various interesting cosmological scenarios. Specifically, for a given range of parameters one avoids the cosmological singularity. We provide a full "phase diagram" of possible Universe evolution for the given parameters. For a certain range of parameters, the thermal averaged Banks-Zaks theory alone results in an exponentially contracting uni- verse followed by a non-singular bounce and an exponentially expanding universe, i.e. Inflation without a Big Bang singularity, or shortly termed "dS Bounce". The tem- perature of such a universe is bounded from above and below. The result is a theory violating the classical Null Energy Condition (NEC). Considering the Banks-Zaks the- ory with an additional perfect fluid, yields an even richer phase diagram that includes the standard Big Bang model, stable single "normal" bounce, dS Bounce and stable cyclic solutions. The bouncing and cyclic solutions are with no singularities, and the violation of the NEC happens only near the bounce. We also provide simple analytical conditions for the existence of these non-singular solutions. Hence, within effective field theory, we have a new alternative non-singular cosmology based on the anoma- arXiv:1912.10532v2 [hep-th] 13 May 2020 lous dimension of Bank-Zaks theory that may include inflation and without resorting to scalar fields. -
Lecture 19 Big Bang Cosmology
PHY100 ― The Nature of the Physical World Lecture 19 Big Bang Cosmology Arán García-Bellido PHY100 1 News Exam 2: you can do better! Presentations April 14: Great Physicist life, Controlled fusion April 19: Nuclear power, Search for ET life April 21: Music, Nuclear terrorism 2 per day 20 min+discussion/Q&A Want to meet with all groups this week Contact me PHY100 2 Cosmology Scientific study of the large scale structure of the universe Attempt to understand the origin, evolution and fate of the universe The universe: all matter, space, time and energy Remember first lecture: Tycho Brahe (1546-1601): careful observation of sun, moon, planets Johannes Kepler (1571-1630): elliptical orbits, 3 laws of planetary motion Isaac Newton (1643-1727): Universal law of gravitation + laws of motion explained Kepler's laws of planetary motion Albert Einstein (1879-1955): In an accelerated rocket ship, light would seem to travel on a curved path Gravity = accelerated frame Gravity curves space PHY100 3 Gravity bends light From outside Light is not bent because of the gravitational force 'per se' Light moves on a geodesic (=shortest distance between two points) So Einstein interprets gravitation as a curvature of spacetime Gravity warps spacetime Light just follows the curvature of space From inside PHY100 4 General Relativity: tests Bending of light by gravitational field 4 Gravitational redshift of light 4 Perihelion advance of Mercury 4 Gravitational waves ? PHY100 5 Light travels at a finite speed Object Lookback time Sun 8 minutes Alpha Centauri -
19. Big-Bang Cosmology 1 19
19. Big-Bang cosmology 1 19. BIG-BANG COSMOLOGY Revised September 2009 by K.A. Olive (University of Minnesota) and J.A. Peacock (University of Edinburgh). 19.1. Introduction to Standard Big-Bang Model The observed expansion of the Universe [1,2,3] is a natural (almost inevitable) result of any homogeneous and isotropic cosmological model based on general relativity. However, by itself, the Hubble expansion does not provide sufficient evidence for what we generally refer to as the Big-Bang model of cosmology. While general relativity is in principle capable of describing the cosmology of any given distribution of matter, it is extremely fortunate that our Universe appears to be homogeneous and isotropic on large scales. Together, homogeneity and isotropy allow us to extend the Copernican Principle to the Cosmological Principle, stating that all spatial positions in the Universe are essentially equivalent. The formulation of the Big-Bang model began in the 1940s with the work of George Gamow and his collaborators, Alpher and Herman. In order to account for the possibility that the abundances of the elements had a cosmological origin, they proposed that the early Universe which was once very hot and dense (enough so as to allow for the nucleosynthetic processing of hydrogen), and has expanded and cooled to its present state [4,5]. In 1948, Alpher and Herman predicted that a direct consequence of this model is the presence of a relic background radiation with a temperature of order a few K [6,7]. Of course this radiation was observed 16 years later as the microwave background radiation [8]. -
Vital Statistics of the Universe the Big Bang So Far…
Vital Statistics of the Universe Today… Observational evidence for the Big Bang Vital statistics of the Universe Hubble’s Constant (H 0) Curvature (k) Density ( Ω) How will it all end? Ho (Expand forever - or collapse?) ΛΛΛ Ω K Our Evolving Universe 1 The Big Bang so far… The universe is expanding & cooling after an initial ‘explosion’ ~14 billion years ago. T = 0 380,000 years Now The CMB… Very hot ~ 3K ‘Inflation’ Matter ‘Recombination’ Structure Particle/photon ‘Freeze-out’ formation Soup ‘Nucleosynthesis ’ Our Evolving Universe 2 PHY111 Our Evolving Universe Lecture 3: Vital Statistics of the Universe Evidence for the Big Bang 1996 type 1 SNe Hubble’s Law × V = H 0 D (H 0=Hubble’s Constant) Evidence for expanding universe (not specifically for Big Bang) The Cosmic Microwave Background Photons from ‘recombination’ at T= 380,000 yrs Evidence for a HOT beginning Our Evolving Universe 3 Evidence for the Big Bang The evolution of galaxies Distant (older) galaxies look different from those nearby. Older galaxies generally smaller, more irregular & more interacting More active galaxies Evidence for changing, non- eternal universe. Earlier lecture ‘Evolution of Galaxies’ Our Evolving Universe 4 PHY111 Our Evolving Universe Lecture 3: Vital Statistics of the Universe Evidence for the Big Bang… The abundance of elements Big Bang Nucleosynthesis predicts: p 4 Mostly Hydrogen & Helium formed - 2H with much smaller quantities of heavier n elements. p 3He n 4He Relative abundance of 4Helium to Hydrogen predicted to be 25% (by mass) of total. Dependent on ratio of proton to neutrons at the +3He (Rare) time of nucleosynthesis (~7:1) 9Be 7 2 3 7 Li Levels of H, He and Li also predicted. -
God, the Multiverse, and Everything
God, the Multiverse, and Everything Rodney Holder CiS Durham, 19.10.2006, 7.30 pm Introduction Sherlock Holmes and Dr Watson went on a camping trip. After a good meal and a bottle of wine they lay down for the night and went to sleep. Some hours later, Holmes awoke and nudged his faithful friend. ‘Watson, look up at the sky and tell me what you see.’ Watson replied, ‘I see millions and millions of stars.’ ‘What does that tell you?’ Watson pondered for a minute. ‘Astronomically, it tells me that there are millions of galaxies and potentially billions of planets. Astrologically, I observe that Saturn is in Leo. Horologically, I deduce that the time is approximately a quarter past three. Theologically, I can see that God is all-powerful and that we are small, and insignificant. Meteorologically, I suspect that we will have a beautiful day tomorrow. What does it tell you, Holmes?’ Holmes was silent for a minute, then spoke. ‘It tells me that some thief has stolen our tent.’ The Big Bang Now you may recognise the person on the right of this photograph. It is of course Einstein himself. The person on the left is less well know but he is one of the truly great figures in cosmology. He is the Abbé George-Henri Lemaître. He was a Belgian priest. I learned only 1 last week that he was in fact a member of my present College, St Edmund’s, in Cambridge. He is reputed to have said, ‘There are two ways to truth and I have chosen them both.’ In 1927 Lemaître solved Einstein’s equations of general relativity for the universe as a whole.