An Investigation of Novel Genetic Tools for the Manipulation of CHO Cell Phenotypes During Recombinant Protein Production
Total Page:16
File Type:pdf, Size:1020Kb
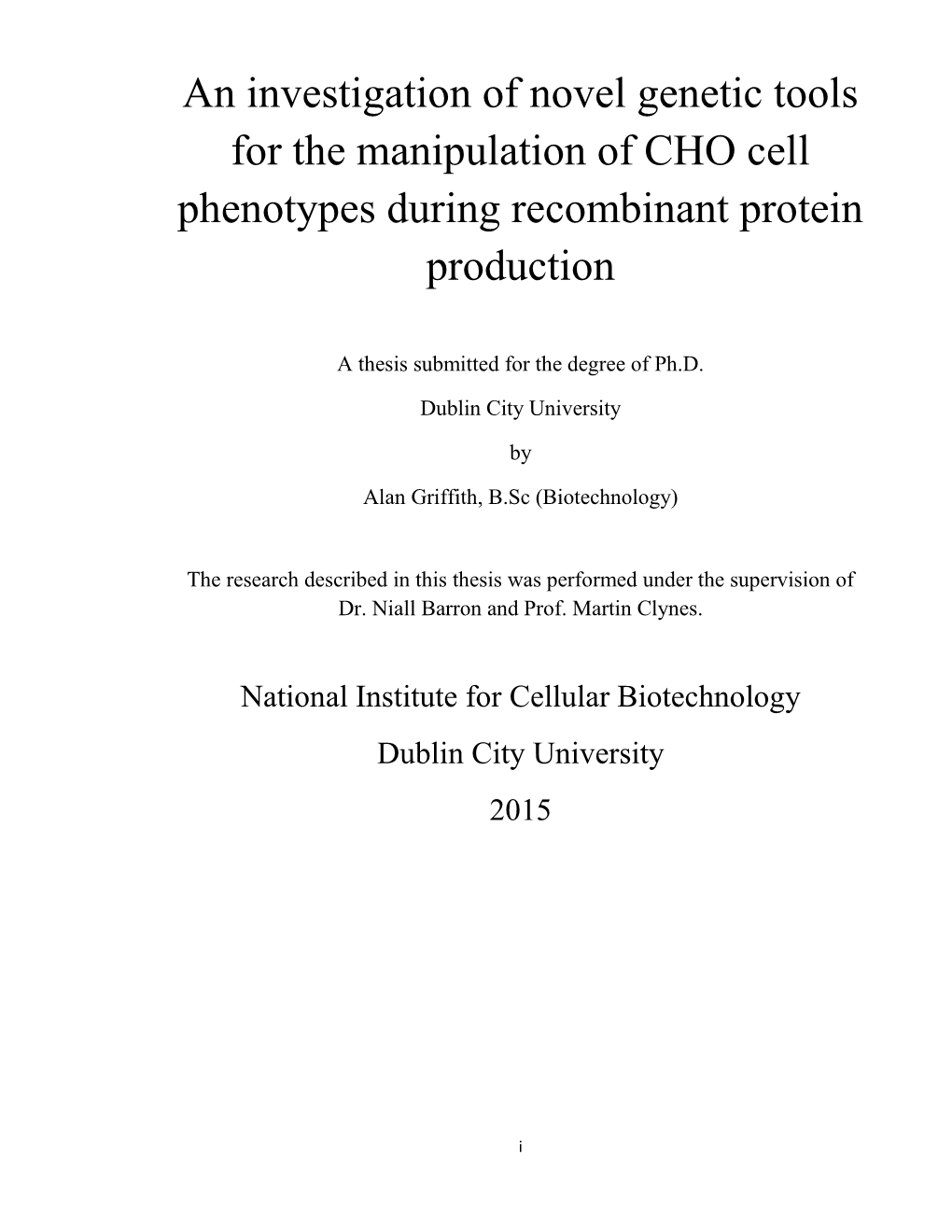
Load more
Recommended publications
-
Upregulation of Peroxisome Proliferator-Activated Receptor-Α And
Upregulation of peroxisome proliferator-activated receptor-α and the lipid metabolism pathway promotes carcinogenesis of ampullary cancer Chih-Yang Wang, Ying-Jui Chao, Yi-Ling Chen, Tzu-Wen Wang, Nam Nhut Phan, Hui-Ping Hsu, Yan-Shen Shan, Ming-Derg Lai 1 Supplementary Table 1. Demographics and clinical outcomes of five patients with ampullary cancer Time of Tumor Time to Age Differentia survival/ Sex Staging size Morphology Recurrence recurrence Condition (years) tion expired (cm) (months) (months) T2N0, 51 F 211 Polypoid Unknown No -- Survived 193 stage Ib T2N0, 2.41.5 58 F Mixed Good Yes 14 Expired 17 stage Ib 0.6 T3N0, 4.53.5 68 M Polypoid Good No -- Survived 162 stage IIA 1.2 T3N0, 66 M 110.8 Ulcerative Good Yes 64 Expired 227 stage IIA T3N0, 60 M 21.81 Mixed Moderate Yes 5.6 Expired 16.7 stage IIA 2 Supplementary Table 2. Kyoto Encyclopedia of Genes and Genomes (KEGG) pathway enrichment analysis of an ampullary cancer microarray using the Database for Annotation, Visualization and Integrated Discovery (DAVID). This table contains only pathways with p values that ranged 0.0001~0.05. KEGG Pathway p value Genes Pentose and 1.50E-04 UGT1A6, CRYL1, UGT1A8, AKR1B1, UGT2B11, UGT2A3, glucuronate UGT2B10, UGT2B7, XYLB interconversions Drug metabolism 1.63E-04 CYP3A4, XDH, UGT1A6, CYP3A5, CES2, CYP3A7, UGT1A8, NAT2, UGT2B11, DPYD, UGT2A3, UGT2B10, UGT2B7 Maturity-onset 2.43E-04 HNF1A, HNF4A, SLC2A2, PKLR, NEUROD1, HNF4G, diabetes of the PDX1, NR5A2, NKX2-2 young Starch and sucrose 6.03E-04 GBA3, UGT1A6, G6PC, UGT1A8, ENPP3, MGAM, SI, metabolism -
2011/058582 Al O
(12) INTERNATIONAL APPLICATION PUBLISHED UNDER THE PATENT COOPERATION TREATY (PCT) (19) World Intellectual Property Organization International Bureau (10) International Publication Number (43) International Publication Date i 1 m 19 May 2011 (19.05.2011) 2011/058582 Al (51) International Patent Classification: Road, Sholinganallur, Chennai 600 119 (IN). CHEN- C07C 259/06 (2006.01) A61P 31/00 (2006.01) NIAPPAN, Vinoth Kumar [IN/IN]; Orchid Research C07D 277/46 (2006.01) A61K 31/426 (2006.01) Laboratories Ltd., R & D Centre: Plot No: 476/14, Old C07D 277/48 (2006.01) A61K 31/55 (2006.01) Mahabalipuram Road, Sholinganallur, Chennai 600 119 C07D 487/08 (2006.01) (IN). GANESAN, Karthikeyan [IN/IN]; Orchid Re search Laboratories Ltd., R & D Centre: Plot No: 476/14, (21) International Application Number: Old Mahabalipuram Road, Sholinganallur, Chennai 600 PCT/IN20 10/000738 119 (IN). NARAYANAN, Shridhar [IN/IN]; Orchid Re (22) International Filing Date: search Laboratories Ltd., R & D Centre: Plot No: 476/14, 12 November 2010 (12.1 1.2010) Old Mahabalipuram Road, Sholinganallur, Chennai 600 119 (IN). (25) Filing Language: English (74) Agent: UDAYAMPALAYAM PALANISAMY, (26) Publication Language: English Senthilkumar; Orchid Chemicals & Pharmaceuticals (30) Priority Data: LTD., R & D Centre: Plot No: 476/14, Old Mahabalipu 2810/CHE/2009 16 November 2009 (16. 11.2009) IN ram Road, Sholinganallur, Chennai 600 119 (IN). (71) Applicant (for all designated States except US): OR¬ (81) Designated States (unless otherwise indicated, for every CHID RESEARCH LABORATORIES LTD. [IN/IN]; kind of national protection available): AE, AG, AL, AM, Orchid Towers, 313, Valluvar Kottam High Road, AO, AT, AU, AZ, BA, BB, BG, BH, BR, BW, BY, BZ, Nungambakkam, Chennai 600 034 (IN). -
Microspore Embryogenesis: Targeting the Determinant Factors of Stress- Induced Cell Reprogramming for Crop Improvement
1 2 3 4 Microspore embryogenesis: targeting the determinant factors of stress- 5 induced cell reprogramming for crop improvement 6 7 8 Pilar S. Testillano 9 10 Pollen Biotechnolgy of Crop Plants group. Biological Research Center, CIB-CSIC. 11 Ramiro de Maeztu 9, 28040 Madrid. Spain 12 E-mail: [email protected] 13 Phone: +34-918373112 (Ext: 4366) 14 15 Date of submission: October 31, 2018 16 Number of figures: 5. 17 Word count: 6312. 18 19 Running title: 20 Determinant factors of stress-induced microspore embryogenesis in crops 1 21 ABSTRACT 22 23 Under stress, isolated microspores are reprogrammed in vitro towards embryogenesis, 24 producing doubled haploid plants, useful biotechnological tools in plant breeding as a 25 source of new genetic variability, fixed in homozygous plants in only one generation. 26 Stress-induced cell death and low rates of cell reprogramming are major factors that 27 reduce the process yield. Knowledge gained in recent years has revealed that 28 microspore embryogenesis initiation and progression involve a complex network of 29 factors, whose roles are not yet well understood. Autophagy and cell-death proteases are 30 crucial players in the response to stress, while cell reprogramming and totipotency 31 acquisition are regulated by hormonal and epigenetic mechanisms. Auxin biosynthesis, 32 transport and action are required for microspore embryogenesis. Initial stages involve 33 DNA hypomethylation, H3K9 demethylation, and H3/H4 acetylation. Cell wall 34 remodelling, with pectin de-methylesterification and AGP expression, is necessary for 35 embryo development. We will review recent findings regarding the determinant factors 36 underlying stress-induced microspore embryogenesis, focusing on the role of 37 autophagy, cell death, auxin, chromatin modifications, and cell wall. -
Epigenetic Malleability at Core Promoter Regulates Tobacco PR-1A
bioRxiv preprint doi: https://doi.org/10.1101/2021.07.24.453639; this version posted July 25, 2021. The copyright holder for this preprint (which was not certified by peer review) is the author/funder. All rights reserved. No reuse allowed without permission. 1 Research article: 2 Epigenetic Malleability at Core Promoter Regulates Tobacco PR-1a 3 Expression after Salicylic Acid Treatment 4 5 Authors: Niraj Lodhi1*, Mala Singh1, Rakesh Srivastava1, Samir V. Sawant1, and Rakesh 6 Tuli1,3 7 8 1. National Botanical Research Institute, Council of Scientific and Industrial Research, Rana 9 Pratap Marg, Lucknow-226001, India 10 2. Present address: University Institute of Engineering & Technology (UIET), Sector 25, 11 Panjab University, Chandigarh 160014, India 12 13 14 *Corresponding Author: 15 Niraj Lodhi, Ph.D. 16 Lead, Clinical Research (Research and Development Division) 17 Genesis DiaGnostics 18 LanGhorne, PA 19047 19 Ph: 267-212-2000 Ext. 156 20 Fax: 267-212-2005 21 E-mail: [email protected] 22 23 24 25 SUMMARY 26 Histone methylation and acetylation regulation of tobacco PR-1a promoter are significant for 27 disassembly of the nucleosome and repressor proteins during induction. 28 1 bioRxiv preprint doi: https://doi.org/10.1101/2021.07.24.453639; this version posted July 25, 2021. The copyright holder for this preprint (which was not certified by peer review) is the author/funder. All rights reserved. No reuse allowed without permission. 29 ABSTRACT 30 • Tobacco’s PR-1a gene is induced by pathogen attack or exogenous application of 31 Salicylic Acid (SA). However, the epigenetic modifications of the most important 32 inducible promoter of the PR-1a gene are not understood clearly. -
Gene Expression-Based Chemical Genomics Identifies Potential Therapeutic Drugs in Hepatocellular Carcinoma
Gene Expression-Based Chemical Genomics Identifies Potential Therapeutic Drugs in Hepatocellular Carcinoma Ming-Huang Chen1., Wu-Lung R. Yang2., Kuan-Ting Lin3,4., Chia-Hung Liu4,5., Yu-Wen Liu1., Kai-Wen Huang6., Peter Mu-Hsin Chang1, Jin-Mei Lai7, Chun-Nan Hsu4,8, Kun-Mao Chao2,5, Cheng-Yan Kao2,5, Chi-Ying F. Huang1,3,9* 1 Institute of Clinical Medicine, National Yang-Ming University, Taipei, Taiwan, 2 Department of Computer Science and Information Engineering, National Taiwan University, Taipei, Taiwan, 3 Institute of Biomedical Informatics, National Yang-Ming University, Taipei, Taiwan, 4 Institute of Information Science, Academia Sinica, Taipei, Taiwan, 5 Graduate Institute of Biomedical Electronic and Bioinformatics, National Taiwan University, Taipei, Taiwan, 6 Department of Surgery & Hepatitis Research Center, National Taiwan University Hospital, National Taiwan University, Taipei, Taiwan, 7 Department of Life Science, Fu-Jen Catholic University, New Taipei City, Taiwan, 8 Information Sciences Institute, University of Southern California, Marina del Rey, California, United States of America, 9 Institute of Biopharmaceutical Sciences, National Yang-Ming University, Taipei, Taiwan Abstract Hepatocellular carcinoma (HCC) is an aggressive tumor with a poor prognosis. Currently, only sorafenib is approved by the FDA for advanced HCC treatment; therefore, there is an urgent need to discover candidate therapeutic drugs for HCC. We hypothesized that if a drug signature could reverse, at least in part, the gene expression signature of HCC, it might have the potential to inhibit HCC-related pathways and thereby treat HCC. To test this hypothesis, we first built an integrative platform, the ‘‘Encyclopedia of Hepatocellular Carcinoma genes Online 2’’, dubbed EHCO2, to systematically collect, organize and compare the publicly available data from HCC studies. -
(ESI) for Integrative Biology. This Journal Is © the Royal Society of Chemistry 2017
Electronic Supplementary Material (ESI) for Integrative Biology. This journal is © The Royal Society of Chemistry 2017 Table 1 Enriched GO terms with p-value ≤ 0.05 corresponding to the over-expressed genes upon perturbation with the lung-toxic compounds. Terms with corrected p-value less than 0.001 are shown in bold. GO:0043067 regulation of programmed GO:0010941 regulation of cell death cell death GO:0042981 regulation of apoptosis GO:0010033 response to organic sub- stance GO:0043068 positive regulation of pro- GO:0010942 positive regulation of cell grammed cell death death GO:0006357 regulation of transcription GO:0043065 positive regulation of apop- from RNA polymerase II promoter tosis GO:0010035 response to inorganic sub- GO:0043066 negative regulation of stance apoptosis GO:0043069 negative regulation of pro- GO:0060548 negative regulation of cell death grammed cell death GO:0016044 membrane organization GO:0042592 homeostatic process GO:0010629 negative regulation of gene ex- GO:0001568 blood vessel development pression GO:0051172 negative regulation of nitrogen GO:0006468 protein amino acid phosphoryla- compound metabolic process tion GO:0070482 response to oxygen levels GO:0045892 negative regulation of transcrip- tion, DNA-dependent GO:0001944 vasculature development GO:0046907 intracellular transport GO:0008202 steroid metabolic process GO:0045934 negative regulation of nucle- obase, nucleoside, nucleotide and nucleic acid metabolic process GO:0006917 induction of apoptosis GO:0016481 negative regulation of transcrip- tion GO:0016125 sterol metabolic process GO:0012502 induction of programmed cell death GO:0001666 response to hypoxia GO:0051253 negative regulation of RNA metabolic process GO:0008203 cholesterol metabolic process GO:0010551 regulation of specific transcrip- tion from RNA polymerase II promoter 1 Table 2 Enriched GO terms with p-value ≤ 0.05 corresponding to the under-expressed genes upon perturbation with the lung-toxic compounds. -
Structure and Function of Metallohydrolases in the Arginase- Deacetylase Family
University of Pennsylvania ScholarlyCommons Publicly Accessible Penn Dissertations 2016 Structure and Function of Metallohydrolases in the Arginase- Deacetylase Family Yang Hai University of Pennsylvania, [email protected] Follow this and additional works at: https://repository.upenn.edu/edissertations Part of the Biochemistry Commons Recommended Citation Hai, Yang, "Structure and Function of Metallohydrolases in the Arginase-Deacetylase Family" (2016). Publicly Accessible Penn Dissertations. 1753. https://repository.upenn.edu/edissertations/1753 This paper is posted at ScholarlyCommons. https://repository.upenn.edu/edissertations/1753 For more information, please contact [email protected]. Structure and Function of Metallohydrolases in the Arginase-Deacetylase Family Abstract Arginases and deacetylases are metallohydrolases that catalyze two distinct chemical transformations. The arginases catalyze the hydrolysis of the guanidinium group of arginine by using a hydroxide ion 2+ 2+ bridging the binuclear manganese cluster (Mn A-Mn B) for nucleophilic attack. The deacetylases catalyze the hydrolysis of amide bonds by using a mononuclear Zn2+-ion activated water molecule as the nucleophile. Despite the diverse functions, metallohydrolases of the arginase-deacetylase superfamily 2+ share the same characteristic α/β hydrolase core fold and a conserved metal binding site (the Mn B site in arginase corresponds to the catalytic Zn2+ site in deacetylase) which is essential for catalysis in both enzymes. We report crystal structure of formiminoglutamase from the parasitic protozoan Trypanosoma cruzi and confirm that formiminoglutamase is a Mn2+-requiring hydrolase that belongs to the arginase- deacetylase superfamily. We also report the crystal structure of an arginase-like protein from Trypanosoma brucei (TbARG) with unknown function. Although its biological role remains enigmatic, the 2+ evolutionarily more conserved Mn B site can be readily restored in TbARG through side-directed mutagenesis. -
Renoprotective Effect of Combined Inhibition of Angiotensin-Converting Enzyme and Histone Deacetylase
BASIC RESEARCH www.jasn.org Renoprotective Effect of Combined Inhibition of Angiotensin-Converting Enzyme and Histone Deacetylase † ‡ Yifei Zhong,* Edward Y. Chen, § Ruijie Liu,*¶ Peter Y. Chuang,* Sandeep K. Mallipattu,* ‡ ‡ † | ‡ Christopher M. Tan, § Neil R. Clark, § Yueyi Deng, Paul E. Klotman, Avi Ma’ayan, § and ‡ John Cijiang He* ¶ *Department of Medicine, Mount Sinai School of Medicine, New York, New York; †Department of Nephrology, Longhua Hospital, Shanghai University of Traditional Chinese Medicine, Shanghai, China; ‡Department of Pharmacology and Systems Therapeutics and §Systems Biology Center New York, Mount Sinai School of Medicine, New York, New York; |Baylor College of Medicine, Houston, Texas; and ¶Renal Section, James J. Peters Veterans Affairs Medical Center, New York, New York ABSTRACT The Connectivity Map database contains microarray signatures of gene expression derived from approximately 6000 experiments that examined the effects of approximately 1300 single drugs on several human cancer cell lines. We used these data to prioritize pairs of drugs expected to reverse the changes in gene expression observed in the kidneys of a mouse model of HIV-associated nephropathy (Tg26 mice). We predicted that the combination of an angiotensin-converting enzyme (ACE) inhibitor and a histone deacetylase inhibitor would maximally reverse the disease-associated expression of genes in the kidneys of these mice. Testing the combination of these inhibitors in Tg26 mice revealed an additive renoprotective effect, as suggested by reduction of proteinuria, improvement of renal function, and attenuation of kidney injury. Furthermore, we observed the predicted treatment-associated changes in the expression of selected genes and pathway components. In summary, these data suggest that the combination of an ACE inhibitor and a histone deacetylase inhibitor could have therapeutic potential for various kidney diseases. -
Emerging Roles for Non-Selenium Containing ER-Resident Glutathione
Biol. Chem. 2021; 402(3): 271–287 Review Katalin Buday and Marcus Conrad* Emerging roles for non-selenium containing ER-resident glutathione peroxidases in cell signaling and disease https://doi.org/10.1515/hsz-2020-0286 Introduction Received August 17, 2020; accepted October 8, 2020; published online October 22, 2020 Maintenance of redox homeostasis is essential for preventing the oxidative damage of biological molecules such as proteins, Abstract: Maintenance of cellular redox control is pivotal lipids and DNA (Poli et al. 2004). The insufficient elimination of for normal cellular functions and cell fate decisions reactive oxygen species (ROS) may trigger cell death and as including cell death. Among the key cellular redox systems such might be a detrimental factor to human health and life- in mammals, the glutathione peroxidase (GPX) family of span (Navarro-Yepes et al. 2014; Valko et al. 2007). Up until proteins is the largest conferring multifaceted functions now, a number of diseases have been directly linked with and affecting virtually all cellular processes. The endo- dysregulated redox homeostasis, including cancer, neurolog- plasmic reticulum (ER)-resident GPXs, designated as GPX7 ical disorders, cardiovascular diseases, obesity and metabolic and GPX8, are the most recently added members of this diseases, as well as aging (Sayre et al. 2001; Valko et al. 2006, family of enzymes. Recent studies have provided exciting 2007). To counterbalance the level of ROS, mammals have insights how both enzymes support critical processes of the evolved a complex ROS scavenging system consisting of ER including oxidative protein folding, maintenance of ER diverseenzymes,suchascatalase(CAT),superoxidedismut- redox control by eliminating H2O2, and preventing palmitic ase (SOD), thioredoxin reductases (TXNRD) and glutathione acid-induced lipotoxicity. -
Application of Connectivity Mapping in Predictive Toxicology Based on Gene
Application of connectivity mapping in predictive toxicology based on gene expression similarity Joshua L. Smalley1, Timothy W. Gant1, and Shu-Dong Zhang1,2 1Medical Research Council Toxicology Unit, University of Leicester, Systems Toxicology Group, Lancaster Road, Leicester, LE1 9HN, UK 2Centre for Cancer Research and Cell Biology (CCRCB), Queen’s University Belfast, Belfast, UK Corresponding author: Shu-Dong Zhang ([email protected]; [email protected]) Keywords: Connectivity mapping; Query gene signature; Reference gene-expression profile; Predictive toxicology Abstract Connectivity mapping is the process of establishing connections between different biological states using gene expression profiles or signatures. There are a number of applications but in toxicology the most pertinent is for understanding mechanisms of toxicity. In its essence the process involves comparing a query gene signature generated as a result of exposure of a biological system to a chemical to those in a database that have been previously derived. In the ideal situation the query gene expression signature is Ulster University's Research Portal characteristic of the event and will be matched to similarprovided by events in the database. Key View metadata, citation and similar papers at core.ac.uk CORE brought to you by criteria are therefore the means of choosing the signature to be matched and the means by which the match is made. In this article we explore these concepts with examples applicable to toxicology. 1 What is connectivity mapping? The concept of connectivity mapping was first introduced by Lamb et al in 2006 (Lamb et al., 2006). It sought to make association between gene expression due to disease state and that due to drug molecules, or similarly between disease state and gene alteration. -
Differentiating Neuroblastoma: a Systematic Review of the Retinoic Acid, Its Derivatives, and Synergistic Interactions
Journal of Personalized Medicine Systematic Review Differentiating Neuroblastoma: A Systematic Review of the Retinoic Acid, Its Derivatives, and Synergistic Interactions Nadiya Bayeva 1,2, Erin Coll 1,2 and Olga Piskareva 1,2,3,* 1 Cancer Bio-Engineering Group, Department of Anatomy and Regenerative Medicine, RCSI University of Medicine and Health Sciences, D02 YN77 Dublin, Ireland; [email protected] (N.B.); [email protected] (E.C.) 2 School of Pharmacy and Biomolecular Sciences, RCSI University of Medicine and Health Sciences, D02 YN77 Dublin, Ireland 3 National Children’s Research Centre, Our Lady’s Children’s Hospital Crumlin, D12 8MGH Dublin, Ireland * Correspondence: [email protected] Abstract: A neuroblastoma (NB) is a solid paediatric tumour arising from undifferentiated neuronal cells. Despite the recent advances in disease management and treatment, it remains one of the leading causes of childhood cancer deaths, thereby necessitating the development of new therapeutic agents and regimens. Retinoic acid (RA), a vitamin A derivative, is a promising agent that can induce differentiation in NB cells. Its isoform, 13-cis RA or isotretinoin, is used in NB therapy; however, its effectiveness is limited to treating a minimal residual disease as maintenance therapy. As such, research focuses on RA derivatives that might increase the anti-NB action or explores the potential synergy between RA and other classes of drugs, such as cellular processes mediators, epigenetic modifiers, and immune modulators. This review summarises the in vitro, in vivo, and clinical data of RA, its derivatives, and synergising compounds, thereby establishing the most promising RA derivatives and combinations of RA for further investigation. -
Benzodiazepine Derivatives Useful in the Treatment of Autoimmune Disorders
(19) TZZ _ Z T (11) EP 2 418 206 A2 (12) EUROPEAN PATENT APPLICATION (43) Date of publication: (51) Int Cl.: 15.02.2012 Bulletin 2012/07 C07D 243/24 (2006.01) C07D 409/12 (2006.01) C07D 403/10 (2006.01) C07D 403/12 (2006.01) (2006.01) (2006.01) (21) Application number: 11188563.8 C07D 405/10 C07D 401/12 A61K 31/55 (2006.01) A61P 35/00 (2006.01) (2006.01) (2006.01) (22) Date of filing: 08.06.2007 A61P 37/00 A61P 29/00 (84) Designated Contracting States: (72) Inventor: Glick, Gary D. AT BE BG CH CY CZ DE DK EE ES FI FR GB GR Ann Arbor, MI Michigan 48103 (US) HU IE IS IT LI LT LU LV MC MT NL PL PT RO SE SI SK TR (74) Representative: Glawe, Delfs, Moll Designated Extension States: Patent- und Rechtsanwälte AL BA HR MK RS Rothenbaumchaussee 58 20148 Hamburg (DE) (30) Priority: 09.06.2006 US 812270 P Remarks: (62) Document number(s) of the earlier application(s) in This application was filed on 10-11-2011 as a accordance with Art. 76 EPC: divisional application to the application mentioned 07795931.0 / 2 037 741 under INID code 62. (71) Applicant: The Regents of the University of Michigan Ann Arbor MI 48109-1280 (US) (54) Benzodiazepine derivatives useful in the treatment of autoimmune disorders (57) The present invention relates to benzodiazepine compounds as therapeutic agents to treat a number of conditions associated with the faulty regulation of the processes of programmed cell death, autoimmunity, in- flammation, hyperproliferation, and the like.