HIV-1 Uncoats in the Nucleus Near Sites of Integration
Total Page:16
File Type:pdf, Size:1020Kb
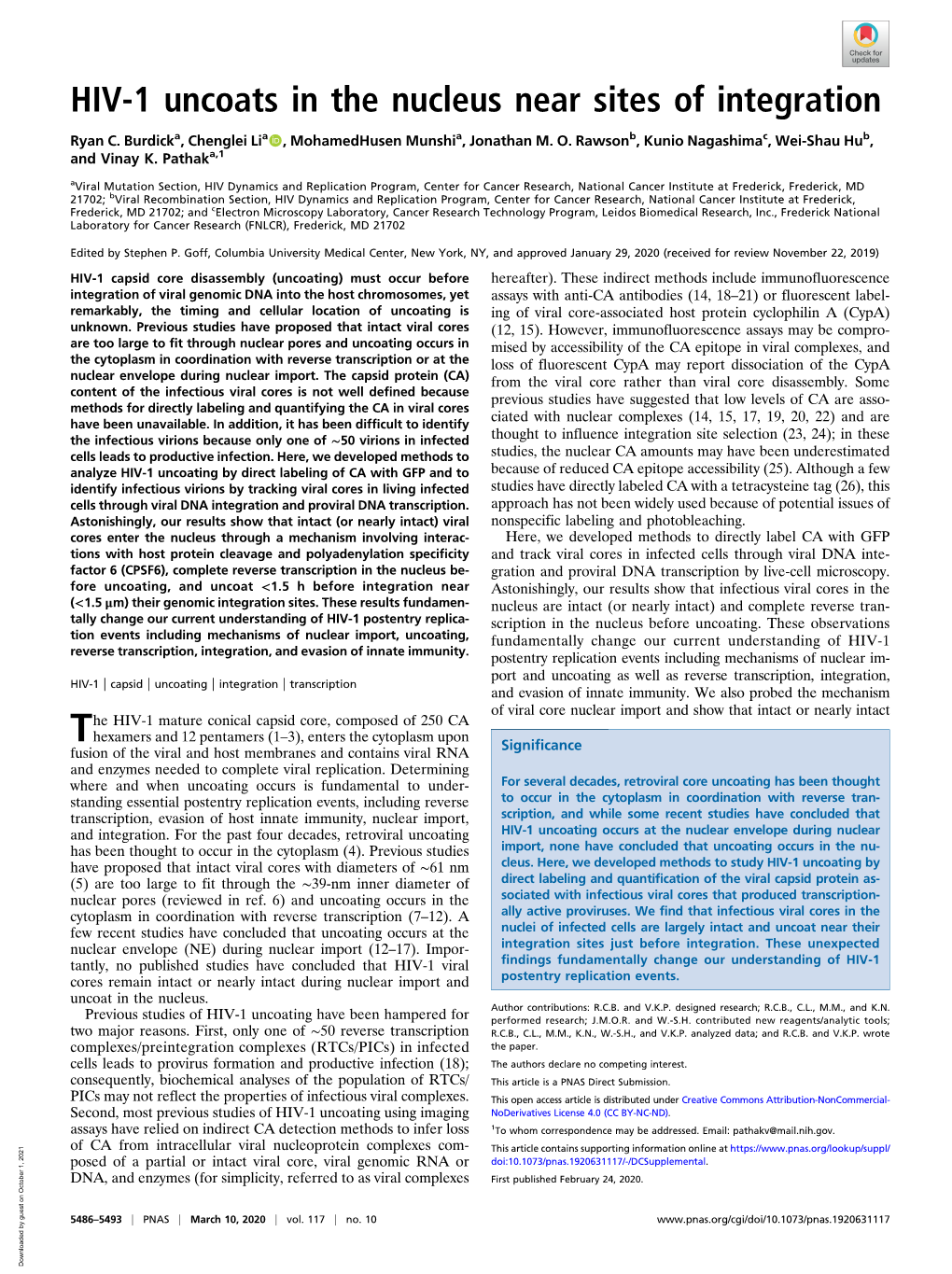
Load more
Recommended publications
-
Multiple Cellular Proteins Interact with LEDGF/P75 Through a Conserved Unstructured Consensus Motif
ARTICLE Received 19 Jan 2015 | Accepted 1 Jul 2015 | Published 6 Aug 2015 DOI: 10.1038/ncomms8968 Multiple cellular proteins interact with LEDGF/p75 through a conserved unstructured consensus motif Petr Tesina1,2,3,*, Katerˇina Cˇerma´kova´4,*, Magdalena Horˇejsˇ´ı3, Katerˇina Procha´zkova´1, Milan Fa´bry3, Subhalakshmi Sharma4, Frauke Christ4, Jonas Demeulemeester4, Zeger Debyser4, Jan De Rijck4,**, Va´clav Veverka1,** & Pavlı´na Rˇeza´cˇova´1,3,** Lens epithelium-derived growth factor (LEDGF/p75) is an epigenetic reader and attractive therapeutic target involved in HIV integration and the development of mixed lineage leukaemia (MLL1) fusion-driven leukaemia. Besides HIV integrase and the MLL1-menin complex, LEDGF/p75 interacts with various cellular proteins via its integrase binding domain (IBD). Here we present structural characterization of IBD interactions with transcriptional repressor JPO2 and domesticated transposase PogZ, and show that the PogZ interaction is nearly identical to the interaction of LEDGF/p75 with MLL1. The interaction with the IBD is maintained by an intrinsically disordered IBD-binding motif (IBM) common to all known cellular partners of LEDGF/p75. In addition, based on IBM conservation, we identify and validate IWS1 as a novel LEDGF/p75 interaction partner. Our results also reveal how HIV integrase efficiently displaces cellular binding partners from LEDGF/p75. Finally, the similar binding modes of LEDGF/p75 interaction partners represent a new challenge for the development of selective interaction inhibitors. 1 Institute of Organic Chemistry and Biochemistry of the ASCR, v.v.i., Flemingovo nam. 2, 166 10 Prague, Czech Republic. 2 Department of Genetics and Microbiology, Faculty of Science, Charles University in Prague, Vinicna 5, 128 44 Prague, Czech Republic. -
Educator Materials Hands-On Activity HIV Reverse Transcription and AZT
Hands-on Activity HIV Reverse Transcription and AZT Educator Materials HIV REVERSE TRANSCRIPTION AND AZT OVERVIEW This hands-on activity is part of a series of activities and demonstrations focusing on various aspects of the human immunodeficiency virus (HIV) life cycle. In this activity, students will model how the anti-HIV drug AZT (azidothymidine) interferes with the process of viral replication and reduces the amount of virus in the Body. Students will first model reverse transcription, the process that results in the production of a double- stranded DNA copy of the HIV single-stranded RNA genome. (See introduction in the student document for a review of the process.) Using an actual HIV RNA sequence as a template, students will model the synthesis of a complementary strand of DNA By attaching nucleotides to one another. Then, students will demonstrate AZT’s effect on this process. They will suBstitute AZT in place of thymidine. AZT lacks a hydroxyl (OH) group that is necessary for suBsequent nucleotides to attach. When AZT is incorporated in a growing DNA sequence it prevents further nucleotides from Being added, thereby blocking the production of HIV DNA. Following this activity, it may Be helpful for students to complete the HIV integration activity. LEARNING OBJECTIVES Students will Be able to: • Code DNA from RNA. • Demonstrate the process of reverse transcription. • Understand the role of the enzyme reverse transcriptase in the formation of viral DNA. • Review the chemical structures of nucleic acids and identify structural -
Proviruses with Long-Term Stable Expression Accumulate In
viruses Article Proviruses with Long-Term Stable Expression Accumulate in Transcriptionally Active Chromatin Close to the Gene Regulatory Elements: Comparison of ASLV-, HIV- and MLV-Derived Vectors Dalibor Miklík 1,2, Filip Šenigl 1 and Jiˇrí Hejnar 1,* 1 Institute of Molecular Genetics, Czech Academy of Sciences, Videnska 1083, CZ-14220 Prague 4, Czech Republic; [email protected] (D.M.); [email protected] (F.S.) 2 Faculty of Science, Charles University, Albertov 6, CZ-12843 Prague 2, Czech Republic * Correspondence: [email protected] Received: 29 January 2018; Accepted: 6 March 2018; Published: 8 March 2018 Abstract: Individual groups of retroviruses and retroviral vectors differ in their integration site preference and interaction with the host genome. Hence, immediately after infection genome-wide distribution of integrated proviruses is non-random. During long-term in vitro or persistent in vivo infection, the genomic position and chromatin environment of the provirus affects its transcriptional activity. Thus, a selection of long-term stably expressed proviruses and elimination of proviruses, which have been gradually silenced by epigenetic mechanisms, helps in the identification of genomic compartments permissive for proviral transcription. We compare here the extent and time course of provirus silencing in single cell clones of the K562 human myeloid lymphoblastoma cell line that have been infected with retroviral reporter vectors derived from avian sarcoma/leukosis virus (ASLV), human immunodeficiency virus type 1 (HIV) and murine leukaemia virus (MLV). While MLV proviruses remain transcriptionally active, ASLV proviruses are prone to rapid silencing. The HIV provirus displays gradual silencing only after an extended time period in culture. -
HIV Integration Site Analysis of Cellular Models of HIV Latency with a Probe-Enriched Next-Generation Sequencing Assay
crossmark HIV Integration Site Analysis of Cellular Models of HIV Latency with a Probe-Enriched Next-Generation Sequencing Assay Sara Sunshine,a Rory Kirchner,b Sami S. Amr,c Leandra Mansur,c Rimma Shakhbatyan,c Michelle Kim,d,e Alberto Bosque,f Robert F. Siliciano,d,e Vicente Planelles,f Oliver Hofmann,b Shannan Ho Sui,b Jonathan Z. Lia Division of Infectious Diseases, Brigham and Women’s Hospital, Harvard Medical School, Boston, Massachusetts, USAa; Bioinformatics Core, Harvard T. H. Chan School of Public Health, Boston, Massachusetts, USAb; Partners HealthCare Personalized Medicine, Cambridge, Massachusetts, USAc; Department of Medicine, Johns Hopkins University School of Medicine, Baltimore, Maryland, USAd; Howard Hughes Medical Institute, Chevy Chase, Maryland, USAe; Department of Pathology, University of Utah, Salt Lake City, Utah, USAf ABSTRACT Downloaded from Antiretroviral therapy (ART) is successful in the suppression of HIV but cannot target and eradicate the latent proviral reservoir. The location of retroviral integration into the human genome is thought to play a role in the clonal expansion of infected cells and HIV persistence. We developed a high-throughput targeted sequence capture assay that uses a pool of HIV-specific probes to enrich Illumina libraries prior to deep sequencing. Using an expanded clonal population of ACH-2 cells, we demonstrate that this sequence capture assay has an extremely low false-positive rate. This assay assessed four cellular models commonly used to -study HIV latency and latency-reversing agents: ACH-2 cells, J-Lat cells, the Bcl-2-transduced primary CD4؉ model, and the cul ؉ tured TCM (central memory) CD4 model. HIV integration site characteristics and genes were compared between these cellular models and to previously reported patient data sets. -
Functional Control of HIV-1 Post-Transcriptional Gene Expression by Host Cell Factors
Functional control of HIV-1 post-transcriptional gene expression by host cell factors DISSERTATION Presented in Partial Fulfillment of the Requirements for the Degree Doctor of Philosophy in the Graduate School of The Ohio State University By Amit Sharma, B.Tech. Graduate Program in Molecular Genetics The Ohio State University 2012 Dissertation Committee Dr. Kathleen Boris-Lawrie, Advisor Dr. Anita Hopper Dr. Karin Musier-Forsyth Dr. Stephen Osmani Copyright by Amit Sharma 2012 Abstract Retroviruses are etiological agents of several human and animal immunosuppressive disorders. They are associated with certain types of cancer and are useful tools for gene transfer applications. All retroviruses encode a single primary transcript that encodes a complex proteome. The RNA genome is reverse transcribed into DNA, integrated into the host genome, and uses host cell factors to transcribe, process and traffic transcripts that encode viral proteins and act as virion precursor RNA, which is packaged into the progeny virions. The functionality of retroviral RNA is governed by ribonucleoprotein (RNP) complexes formed by host RNA helicases and other RNA- binding proteins. The 5’ leader of retroviral RNA undergoes alternative inter- and intra- molecular RNA-RNA and RNA-protein interactions to complete multiple steps of the viral life cycle. Retroviruses do not encode any RNA helicases and are dependent on host enzymes and RNA chaperones. Several members of the host RNA helicase superfamily are necessary for progressive steps during the retroviral replication. RNA helicase A (RHA) interacts with the redundant structural elements in the 5’ untranslated region (UTR) of retroviral and selected cellular mRNAs and this interaction is necessary to facilitate polyribosome formation and productive protein synthesis. -
The Role of Integration and Clonal Expansion in HIV Infection: Live Long and Prosper Elizabeth M
Anderson and Maldarelli Retrovirology (2018) 15:71 https://doi.org/10.1186/s12977-018-0448-8 Retrovirology REVIEW Open Access The role of integration and clonal expansion in HIV infection: live long and prosper Elizabeth M. Anderson and Frank Maldarelli* Abstract Integration of viral DNA into the host genome is a central event in the replication cycle and the pathogenesis of retroviruses, including HIV. Although most cells infected with HIV are rapidly eliminated in vivo, HIV also infects long-lived cells that persist during combination antiretroviral therapy (cART). Cells with replication competent HIV proviruses form a reservoir that persists despite cART and such reservoirs are at the center of eforts to eradicate or control infection without cART. The mechanisms of persistence of these chronically infected long-lived cells is uncer- tain, but recent research has demonstrated that the presence of the HIV provirus has enduring efects on infected cells. Cells with integrated proviruses may persist for many years, undergo clonal expansion, and produce replication competent HIV. Even proviruses with defective genomes can produce HIV RNA and may contribute to ongoing HIV pathogenesis. New analyses of HIV infected cells suggest that over time on cART, there is a shift in the composition of the population of HIV infected cells, with the infected cells that persist over prolonged periods having proviruses integrated in genes associated with regulation of cell growth. In several cases, strong evidence indicates the presence of the provirus in specifc genes may determine persistence, proliferation, or both. These data have raised the intrigu- ing possibility that after cART is introduced, a selection process enriches for cells with proviruses integrated in genes associated with cell growth regulation. -
Constrained Mutational Sampling of Amino Acids in HIV-1 Protease Evolution
bioRxiv preprint doi: https://doi.org/10.1101/354597; this version posted January 28, 2019. The copyright holder for this preprint (which was not certified by peer review) is the author/funder, who has granted bioRxiv a license to display the preprint in perpetuity. It is made available under aCC-BY-NC 4.0 International license. Constrained mutational sampling of amino acids in HIV-1 protease evolution Jeffrey I. Boucher1,*, Troy W. Whitfield2,3,*, Ann Dauphin4, Gily Nachum1, Carl Hollins III1, Konstantin B. Zeldovich4, Ronald Swanstrom5, Celia A. Schiffer1, Jeremy Luban2,4, Daniel N. A. Bolon1,# 1Department of Biochemistry and Molecular Pharmacology, 2Department of Medicine, 3Program in Bioinformatics and Integrative Biology, 4Program in Molecular Medicine, University of Massachusetts Medical School, Worcester, MA 01605, USA 5Department of Biochemistry and Biophysics, University of North Carolina, Chapel Hill, NC USA *Co-first authors #Corresponding Author bioRxiv preprint doi: https://doi.org/10.1101/354597; this version posted January 28, 2019. The copyright holder for this preprint (which was not certified by peer review) is the author/funder, who has granted bioRxiv a license to display the preprint in perpetuity. It is made available under aCC-BY-NC 4.0 International license. Abstract The evolution of HIV-1 protein sequences should be governed by a combination of factors including nucleotide mutational probabilities, the genetic code, and fitness. The impact of these factors on protein sequence evolution are interdependent, making it challenging to infer the individual contribution of each factor from phylogenetic analyses alone. We investigated the protein sequence evolution of HIV-1 by determining an experimental fitness landscape of all individual amino acid changes in protease. -
Disruption of the Terminal Base Pairs of Retroviral DNA During Integration
Downloaded from genesdev.cshlp.org on September 25, 2021 - Published by Cold Spring Harbor Laboratory Press Disruption of the terminal base pairs of retroviral DNA during integration Brian P. Scottoline/ Samson Chow,^"* Viola Ellison/'^ and Patrick O. Brown^ 2,5 ^Department of Biochemistry, and ^Howard Hughes Medical Institute, Stanford University Medical School, Stanford, California 94305-5428 USA Integrase catalyzes two essential steps in the integration of the retroviral genome—end processing and strand transfer—both of which require the interaction of integrase with viral att sites located at the ends of viral genomic DNA. These two different polynucleotidyl transfer reactions are apparently carried out by a single active site. The end product of these reactions, the integrated provirus, does not undergo transposition and remains a stable part of the host cell genome. A central question in understanding the mechanism of integration is how a single active site accomplishes two distinct polynucleotidyl transfer reactions. We propose that integrase distorts DNA substrates to accommodate both reactions within the active site. Evidence is provided for disruption of base-pairing at the terminus of viral DNA during end processing. Furthermore, we show that this end fraying is a required step in end processing and that it appears to occur after initial binding of the viral DNA end. This requirement for base-pair disruption may account for the inability of integrase to use internal sites on DNA molecules as viral att sites. The specificity of integrase for DNA ends solves a problem posed by the long terminal repeat structure of the viral genome, and may help to prevent transposition of integrated proviruses. -
LEDGF/P75 Functions Downstream from Preintegration Complex Formation to Effect Gene-Specific HIV-1 Integration
Downloaded from genesdev.cshlp.org on October 1, 2021 - Published by Cold Spring Harbor Laboratory Press LEDGF/p75 functions downstream from preintegration complex formation to effect gene-specific HIV-1 integration Ming-Chieh Shun,1,4 Nidhanapati K. Raghavendra,1,4 Nick Vandegraaff,1,4,5 Janet E. Daigle,1 Siobhan Hughes,2 Paul Kellam,3 Peter Cherepanov,2,7 and Alan Engelman1,6 1Department of Cancer Immunology and AIDS, Dana-Farber Cancer Institute, Division of AIDS, Harvard Medical School, Boston, Massachusetts 02115, USA; 2Division of Medicine, Imperial College London, St. Mary’s Campus, London W2 1PG, United Kingdom; 3Department of Infection, University College London, London W1T 4JF, United Kingdom LEDGF/p75 directly interacts with lentiviral integrase proteins and can modulate their enzymatic activities and chromosomal association. A novel genetic knockout model was established that allowed us for the first time to analyze HIV-1 integration in the absence of LEDGF/p75 protein. Supporting a crucial role for the cofactor in viral replication, HIV-1 vector integration and reporter gene expression were significantly reduced in LEDGF-null cells. Yet, integrase processed the viral cDNA termini normally and maintained its local target DNA sequence preference during integration. Preintegration complexes extracted from knockout cells moreover supported normal levels of DNA strand transfer activity in vitro. In contrast, HIV-1 lost its strong bias toward integrating into transcription units, displaying instead increased affinity for promoter regions and CpG islands. Our results reveal LEDGF/p75 as a critical targeting factor, commandeering lentiviruses from promoter- and/or CpG island-proximal pathways that are favored by other members of Retroviridae. -
HIV-1 Integrase-DNA Recognition Mechanisms
Viruses 2009, 1, 713-736; doi:10.3390/v1030713 OPEN ACCESS viruses ISSN 1999-4915 www.mdpi.com/journal/viruses Review HIV-1 Integrase-DNA Recognition Mechanisms Jacques J. Kessl, Christopher J. McKee, Jocelyn O. Eidahl, Nikolozi Shkriabai, Ari Katz and Mamuka Kvaratskhelia * Center for Retrovirus Research and Comprehensive Cancer Center, College of Pharmacy, The Ohio State University, Columbus, OH 43210, USA; E-Mails: [email protected] (J.J.K.); [email protected] (C.J.M.); [email protected] (J.O.E.), [email protected] (N.S.); [email protected] (A.K.) * Author to whom correspondence should be addressed; E-Mail: [email protected]; Tel.: +1-614-292-6091; Fax: +1-614-292-7766. Received: 30 September 2009; in revised form: 3 November 2009 / Accepted: 4 November 2009 / Published: 5 November 2009 Abstract: Integration of a reverse transcribed DNA copy of the HIV viral genome into the host chromosome is essential for virus replication. This process is catalyzed by the virally encoded protein integrase. The catalytic activities, which involve DNA cutting and joining steps, have been recapitulated in vitro using recombinant integrase and synthetic DNA substrates. Biochemical and biophysical studies of these model reactions have been pivotal in advancing our understanding of mechanistic details for how IN interacts with viral and target DNAs, and are the focus of the present review. Keywords: HIV; retroviruses; DNA; integrase; crosslinking; footprinting 1. Introduction HIV-1 integrase (IN) catalyses integration of the reverse transcribed DNA copy of the viral genome into a host chromosome (reviewed in [1]), a step which is essential for the retroviral lifecycle. -
The Emerging Role of HIV-1 Integrase in Virion Morphogenesis
viruses Review Going beyond Integration: The Emerging Role of HIV-1 Integrase in Virion Morphogenesis Jennifer L. Elliott and Sebla B. Kutluay * Department of Molecular Microbiology, Washington University School of Medicine, Saint Louis, MO 63110, USA; [email protected] * Correspondence: [email protected] Received: 26 August 2020; Accepted: 7 September 2020; Published: 9 September 2020 Abstract: The HIV-1 integrase enzyme (IN) plays a critical role in the viral life cycle by integrating the reverse-transcribed viral DNA into the host chromosome. This function of IN has been well studied, and the knowledge gained has informed the design of small molecule inhibitors that now form key components of antiretroviral therapy regimens. Recent discoveries unveiled that IN has an under-studied yet equally vital second function in human immunodeficiency virus type 1 (HIV-1) replication. This involves IN binding to the viral RNA genome in virions, which is necessary for proper virion maturation and morphogenesis. Inhibition of IN binding to the viral RNA genome results in mislocalization of the viral genome inside the virus particle, and its premature exposure and degradation in target cells. The roles of IN in integration and virion morphogenesis share a number of common elements, including interaction with viral nucleic acids and assembly of higher-order IN multimers. Herein we describe these two functions of IN within the context of the HIV-1 life cycle, how IN binding to the viral genome is coordinated by the major structural protein, Gag, and discuss the value of targeting the second role of IN in virion morphogenesis. Keywords: HIV-1; integrase; maturation; integrase–RNA interactions; protein–RNA interactions 1. -
HIV-1 Integrase Inhibitors: Update and Perspectives Elena Semenova, Christop
Running title: Targeting HIV Integration Title: HIV-1 Integrase Inhibitors: Update and Perspectives Elena Semenova, Christophe Marchand and Yves Pommier Laboratory of Molecular Pharmacology, Center for Cancer Research, National Cancer Institute, National Institutes of Health, Bethesda, MD 20892-4255. Corresponding author: Yves Pommier; Bldg. 37, Rm. 5068, NIH, Bethesda, MD 20892-4255; [email protected] 1 Abstract HIV replication requires the insertion of the viral genome inside the nuclear genome of infected cells through a recombination process catalyzed by the virus-encoded enzyme, integrase. HIV integrase has recently been recognized as a reachable antiviral target following the promising results of integrase inhibitors in clinical trials. The present review focuses on the recent advances in understanding the cellular mechanisms of HIV integration and the sites of actions of inhibitors. It also provides an extensive list of the known mutations that have been characterized for HIV-1 integrase with their impact on integrase activity, viral replication and response to anti-integrase drugs. Novel rational approaches for inhibiting HIV integration are also discussed, as well as the two integrase inhibitors in clinical trials and other selected inhibitors in development. 2 Outline of the chapter: I. Foreword. II. Integration: a Crucial Step in the HIV Life Cycle. A. HIV-1 Integrase Structure. B. Chemistry of Retroviral Integration. C. Integration Occurs within a Large Macromolecular Complex. III. Approaches to Inhibit HIV Integration. A. Small Molecule inhibitors of HIV Integrase Enzymatic Activities. B. Targeting the Preintegration Complex (PIC). IV. Inhibitors in Clinical Trials. V. Inhibitors in Preclinical Development. VI. Perspectives. 3 I. Foreword The encouraging results reported for two integrase inhibitors in clinical trials and the recent insights in the cellular cofactors of DNA integration, have renewed interest in HIV integrase pharmacology and cellular biology.