SP1 Dynamics-Supplemental
Total Page:16
File Type:pdf, Size:1020Kb
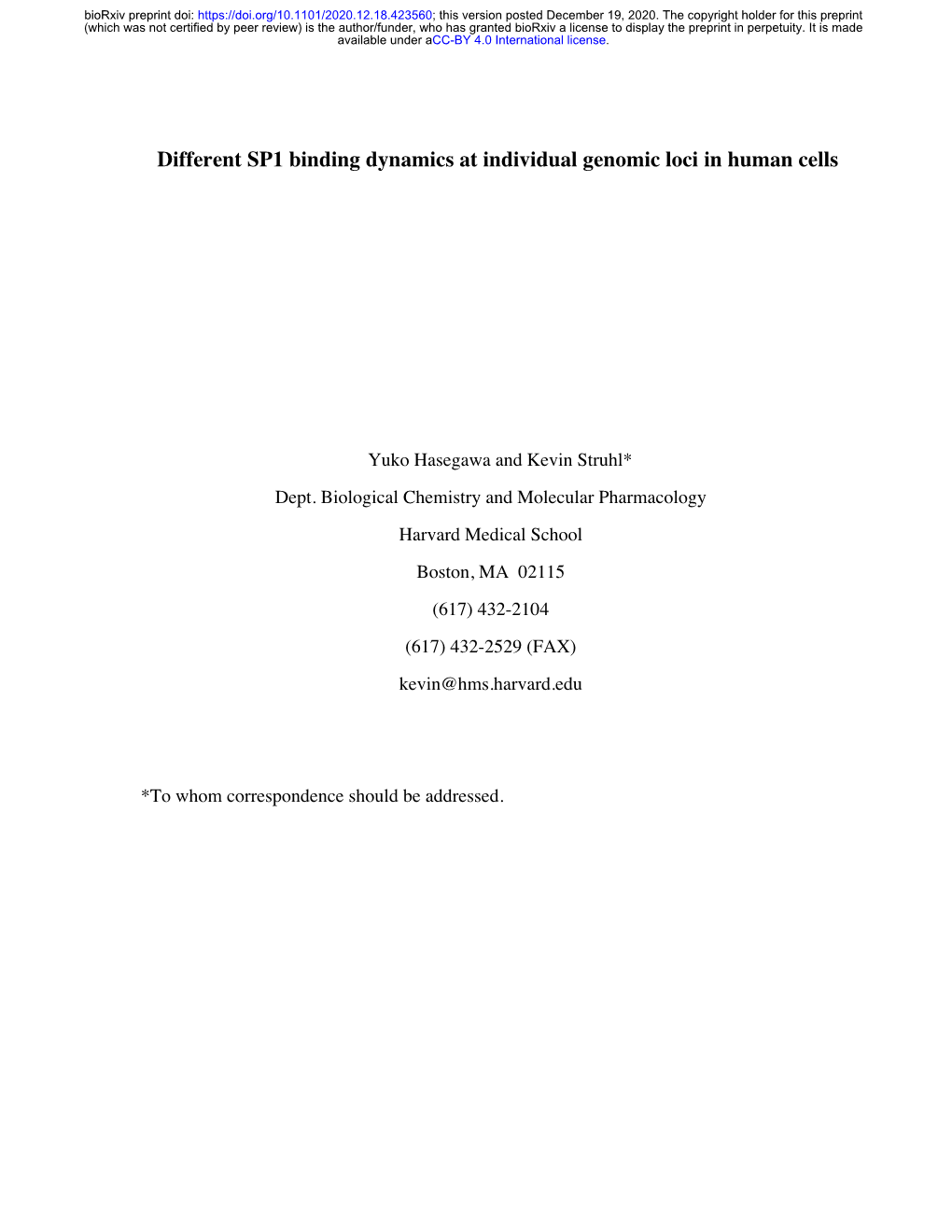
Load more
Recommended publications
-
Down-Regulation of Stem Cell Genes, Including Those in a 200-Kb Gene Cluster at 12P13.31, Is Associated with in Vivo Differentiation of Human Male Germ Cell Tumors
Research Article Down-Regulation of Stem Cell Genes, Including Those in a 200-kb Gene Cluster at 12p13.31, Is Associated with In vivo Differentiation of Human Male Germ Cell Tumors James E. Korkola,1 Jane Houldsworth,1,2 Rajendrakumar S.V. Chadalavada,1 Adam B. Olshen,3 Debbie Dobrzynski,2 Victor E. Reuter,4 George J. Bosl,2 and R.S.K. Chaganti1,2 1Cell Biology Program and Departments of 2Medicine, 3Epidemiology and Biostatistics, and 4Pathology, Memorial Sloan-Kettering Cancer Center, New York, New York Abstract on the degree and type of differentiation (i.e., seminomas, which Adult male germ cell tumors (GCTs) comprise distinct groups: resemble undifferentiated primitive germ cells, and nonseminomas, seminomas and nonseminomas, which include pluripotent which show varying degrees of embryonic and extraembryonic embryonal carcinomas as well as other histologic subtypes patterns of differentiation; refs. 2, 3). Nonseminomatous GCTs are exhibiting various stages of differentiation. Almost all GCTs further subdivided into embryonal carcinomas, which show early show 12p gain, but the target genes have not been clearly zygotic or embryonal-like differentiation, yolk sac tumors and defined. To identify 12p target genes, we examined Affymetrix choriocarcinomas, which exhibit extraembryonal forms of differ- (Santa Clara, CA) U133A+B microarray (f83% coverage of 12p entiation, and teratomas, which show somatic differentiation along genes) expression profiles of 17 seminomas, 84 nonseminoma multiple lineages (3). Both seminomas and embryonal carcinoma GCTs, and 5 normal testis samples. Seventy-three genes on 12p are known to express stem cell markers, such as POU5F1 (4) and were significantly overexpressed, including GLUT3 and REA NANOG (5). -
SUMO-1 Regulates the Conformational Dynamics of Thymine-DNA
Smet-Nocca et al. BMC Biochemistry 2011, 12:4 http://www.biomedcentral.com/1471-2091/12/4 RESEARCHARTICLE Open Access SUMO-1 regulates the conformational dynamics of Thymine-DNA Glycosylase regulatory domain and competes with its DNA binding activity Caroline Smet-Nocca1, Jean-Michel Wieruszeski2, Hélène Léger1,3, Sebastian Eilebrecht1,3, Arndt Benecke1,3* Abstract Background: The human thymine-DNA glycosylase (TDG) plays a dual role in base excision repair of G:U/T mismatches and in transcription. Regulation of TDG activity by SUMO-1 conjugation was shown to act on both functions. Furthermore, TDG can interact with SUMO-1 in a non-covalent manner. Results: Using NMR spectroscopy we have determined distinct conformational changes in TDG upon either covalent sumoylation on lysine 330 or intermolecular SUMO-1 binding through a unique SUMO-binding motif (SBM) localized in the C-terminal region of TDG. The non-covalent SUMO-1 binding induces a conformational change of the TDG amino-terminal regulatory domain (RD). Such conformational dynamics do not exist with covalent SUMO-1 attachment and could potentially play a broader role in the regulation of TDG functions for instance during transcription. Both covalent and non-covalent processes activate TDG G:U repair similarly. Surprisingly, despite a dissociation of the SBM/SUMO-1 complex in presence of a DNA substrate, SUMO-1 preserves its ability to stimulate TDG activity indicating that the non-covalent interactions are not directly involved in the regulation of TDG activity. SUMO-1 instead acts, as demonstrated here, indirectly by competing with the regulatory domain of TDG for DNA binding. -
Molecular Profile of Tumor-Specific CD8+ T Cell Hypofunction in a Transplantable Murine Cancer Model
Downloaded from http://www.jimmunol.org/ by guest on September 25, 2021 T + is online at: average * The Journal of Immunology , 34 of which you can access for free at: 2016; 197:1477-1488; Prepublished online 1 July from submission to initial decision 4 weeks from acceptance to publication 2016; doi: 10.4049/jimmunol.1600589 http://www.jimmunol.org/content/197/4/1477 Molecular Profile of Tumor-Specific CD8 Cell Hypofunction in a Transplantable Murine Cancer Model Katherine A. Waugh, Sonia M. Leach, Brandon L. Moore, Tullia C. Bruno, Jonathan D. Buhrman and Jill E. Slansky J Immunol cites 95 articles Submit online. Every submission reviewed by practicing scientists ? is published twice each month by Receive free email-alerts when new articles cite this article. Sign up at: http://jimmunol.org/alerts http://jimmunol.org/subscription Submit copyright permission requests at: http://www.aai.org/About/Publications/JI/copyright.html http://www.jimmunol.org/content/suppl/2016/07/01/jimmunol.160058 9.DCSupplemental This article http://www.jimmunol.org/content/197/4/1477.full#ref-list-1 Information about subscribing to The JI No Triage! Fast Publication! Rapid Reviews! 30 days* Why • • • Material References Permissions Email Alerts Subscription Supplementary The Journal of Immunology The American Association of Immunologists, Inc., 1451 Rockville Pike, Suite 650, Rockville, MD 20852 Copyright © 2016 by The American Association of Immunologists, Inc. All rights reserved. Print ISSN: 0022-1767 Online ISSN: 1550-6606. This information is current as of September 25, 2021. The Journal of Immunology Molecular Profile of Tumor-Specific CD8+ T Cell Hypofunction in a Transplantable Murine Cancer Model Katherine A. -
2017.08.28 Anne Barry-Reidy Thesis Final.Pdf
REGULATION OF BOVINE β-DEFENSIN EXPRESSION THIS THESIS IS SUBMITTED TO THE UNIVERSITY OF DUBLIN FOR THE DEGREE OF DOCTOR OF PHILOSOPHY 2017 ANNE BARRY-REIDY SCHOOL OF BIOCHEMISTRY & IMMUNOLOGY TRINITY COLLEGE DUBLIN SUPERVISORS: PROF. CLIONA O’FARRELLY & DR. KIERAN MEADE TABLE OF CONTENTS DECLARATION ................................................................................................................................. vii ACKNOWLEDGEMENTS ................................................................................................................... viii ABBREVIATIONS ................................................................................................................................ix LIST OF FIGURES............................................................................................................................. xiii LIST OF TABLES .............................................................................................................................. xvii ABSTRACT ........................................................................................................................................xix Chapter 1 Introduction ........................................................................................................ 1 1.1 Antimicrobial/Host-defence peptides ..................................................................... 1 1.2 Defensins................................................................................................................. 1 1.3 β-defensins ............................................................................................................. -
Chicken CCDC152 Shares an NFYB-Regulated Bidirectional Promoter with a Growth Hormone Receptor Antisense Transcript and Inhibits Cells Proliferation and Migration
www.impactjournals.com/oncotarget/ Oncotarget, 2017, Vol. 8, (No. 48), pp: 84039-84053 Research Paper Chicken CCDC152 shares an NFYB-regulated bidirectional promoter with a growth hormone receptor antisense transcript and inhibits cells proliferation and migration Shudai Lin1, Wei Luo1, Mingya Jiang1, Wen Luo1, Bahareldin Ali Abdalla1, Qinghua Nie1, Li Zhang2 and Xiquan Zhang1 1Guangdong Provincial Key Lab of Agro-Animal Genomics and Molecular Breeding and Key Lab of Chicken Genetics, Breeding and Reproduction, Ministry of Agriculture, College of Animal Science of South China Agricultural University, Guangzhou 510642, P.R. China 2Agricultural College, Guangdong Ocean University, Zhanjiang 524088, P.R. China Correspondence to: Xiquan Zhang, email: [email protected] Li Zhang, email: [email protected] Keywords: bidirectional promoter, GHR antisense transcript, coiled-coil domain containing 152, NFYB, cell cycle Received: December 15, 2016 Accepted: September 04, 2017 Published: September 20, 2017 Copyright: Lin et al. This is an open-access article distributed under the terms of the Creative Commons Attribution License 3.0 (CC BY 3.0), which permits unrestricted use, distribution, and reproduction in any medium, provided the original author and source are credited. ABSTRACT The chicken coiled-coil domain-containing protein 152 (CCDC152) recently has been identified as a novel one implicated in cell cycle regulation, cellular proliferation and migration by us. Here we demonstrate that CCDC152 is oriented in a head-to- head configuration with the antisense transcript of growth hormone receptor (GHR) gene. Through serial luciferase reporter assays, we firstly identified a minimal 102 bp intergenic region as a core bidirectional promoter to drive basal transcription in divergent orientations. -
Mediator of DNA Damage Checkpoint 1 (MDC1) Is a Novel Estrogen Receptor Co-Regulator in Invasive 6 Lobular Carcinoma of the Breast 7 8 Evelyn K
bioRxiv preprint doi: https://doi.org/10.1101/2020.12.16.423142; this version posted December 16, 2020. The copyright holder for this preprint (which was not certified by peer review) is the author/funder, who has granted bioRxiv a license to display the preprint in perpetuity. It is made available under aCC-BY-NC 4.0 International license. 1 Running Title: MDC1 co-regulates ER in ILC 2 3 Research article 4 5 Mediator of DNA damage checkpoint 1 (MDC1) is a novel estrogen receptor co-regulator in invasive 6 lobular carcinoma of the breast 7 8 Evelyn K. Bordeaux1+, Joseph L. Sottnik1+, Sanjana Mehrotra1, Sarah E. Ferrara2, Andrew E. Goodspeed2,3, James 9 C. Costello2,3, Matthew J. Sikora1 10 11 +EKB and JLS contributed equally to this project. 12 13 Affiliations 14 1Dept. of Pathology, University of Colorado Anschutz Medical Campus 15 2Biostatistics and Bioinformatics Shared Resource, University of Colorado Comprehensive Cancer Center 16 3Dept. of Pharmacology, University of Colorado Anschutz Medical Campus 17 18 Corresponding author 19 Matthew J. Sikora, PhD.; Mail Stop 8104, Research Complex 1 South, Room 5117, 12801 E. 17th Ave.; Aurora, 20 CO 80045. Tel: (303)724-4301; Fax: (303)724-3712; email: [email protected]. Twitter: 21 @mjsikora 22 23 Authors' contributions 24 MJS conceived of the project. MJS, EKB, and JLS designed and performed experiments. JLS developed models 25 for the project. EKB, JLS, SM, and AEG contributed to data analysis and interpretation. SEF, AEG, and JCC 26 developed and performed informatics analyses. MJS wrote the draft manuscript; all authors read and revised the 27 manuscript and have read and approved of this version of the manuscript. -
A Computational Approach for Defining a Signature of Β-Cell Golgi Stress in Diabetes Mellitus
Page 1 of 781 Diabetes A Computational Approach for Defining a Signature of β-Cell Golgi Stress in Diabetes Mellitus Robert N. Bone1,6,7, Olufunmilola Oyebamiji2, Sayali Talware2, Sharmila Selvaraj2, Preethi Krishnan3,6, Farooq Syed1,6,7, Huanmei Wu2, Carmella Evans-Molina 1,3,4,5,6,7,8* Departments of 1Pediatrics, 3Medicine, 4Anatomy, Cell Biology & Physiology, 5Biochemistry & Molecular Biology, the 6Center for Diabetes & Metabolic Diseases, and the 7Herman B. Wells Center for Pediatric Research, Indiana University School of Medicine, Indianapolis, IN 46202; 2Department of BioHealth Informatics, Indiana University-Purdue University Indianapolis, Indianapolis, IN, 46202; 8Roudebush VA Medical Center, Indianapolis, IN 46202. *Corresponding Author(s): Carmella Evans-Molina, MD, PhD ([email protected]) Indiana University School of Medicine, 635 Barnhill Drive, MS 2031A, Indianapolis, IN 46202, Telephone: (317) 274-4145, Fax (317) 274-4107 Running Title: Golgi Stress Response in Diabetes Word Count: 4358 Number of Figures: 6 Keywords: Golgi apparatus stress, Islets, β cell, Type 1 diabetes, Type 2 diabetes 1 Diabetes Publish Ahead of Print, published online August 20, 2020 Diabetes Page 2 of 781 ABSTRACT The Golgi apparatus (GA) is an important site of insulin processing and granule maturation, but whether GA organelle dysfunction and GA stress are present in the diabetic β-cell has not been tested. We utilized an informatics-based approach to develop a transcriptional signature of β-cell GA stress using existing RNA sequencing and microarray datasets generated using human islets from donors with diabetes and islets where type 1(T1D) and type 2 diabetes (T2D) had been modeled ex vivo. To narrow our results to GA-specific genes, we applied a filter set of 1,030 genes accepted as GA associated. -
Supplemental Materials ZNF281 Enhances Cardiac Reprogramming
Supplemental Materials ZNF281 enhances cardiac reprogramming by modulating cardiac and inflammatory gene expression Huanyu Zhou, Maria Gabriela Morales, Hisayuki Hashimoto, Matthew E. Dickson, Kunhua Song, Wenduo Ye, Min S. Kim, Hanspeter Niederstrasser, Zhaoning Wang, Beibei Chen, Bruce A. Posner, Rhonda Bassel-Duby and Eric N. Olson Supplemental Table 1; related to Figure 1. Supplemental Table 2; related to Figure 1. Supplemental Table 3; related to the “quantitative mRNA measurement” in Materials and Methods section. Supplemental Table 4; related to the “ChIP-seq, gene ontology and pathway analysis” and “RNA-seq” and gene ontology analysis” in Materials and Methods section. Supplemental Figure S1; related to Figure 1. Supplemental Figure S2; related to Figure 2. Supplemental Figure S3; related to Figure 3. Supplemental Figure S4; related to Figure 4. Supplemental Figure S5; related to Figure 6. Supplemental Table S1. Genes included in human retroviral ORF cDNA library. Gene Gene Gene Gene Gene Gene Gene Gene Symbol Symbol Symbol Symbol Symbol Symbol Symbol Symbol AATF BMP8A CEBPE CTNNB1 ESR2 GDF3 HOXA5 IL17D ADIPOQ BRPF1 CEBPG CUX1 ESRRA GDF6 HOXA6 IL17F ADNP BRPF3 CERS1 CX3CL1 ETS1 GIN1 HOXA7 IL18 AEBP1 BUD31 CERS2 CXCL10 ETS2 GLIS3 HOXB1 IL19 AFF4 C17ORF77 CERS4 CXCL11 ETV3 GMEB1 HOXB13 IL1A AHR C1QTNF4 CFL2 CXCL12 ETV7 GPBP1 HOXB5 IL1B AIMP1 C21ORF66 CHIA CXCL13 FAM3B GPER HOXB6 IL1F3 ALS2CR8 CBFA2T2 CIR1 CXCL14 FAM3D GPI HOXB7 IL1F5 ALX1 CBFA2T3 CITED1 CXCL16 FASLG GREM1 HOXB9 IL1F6 ARGFX CBFB CITED2 CXCL3 FBLN1 GREM2 HOXC4 IL1F7 -
T-Cell Receptor (TCR) Signaling Promotes the Assembly of Ranbp2
RESEARCH ARTICLE T-cell receptor (TCR) signaling promotes the assembly of RanBP2/RanGAP1- SUMO1/Ubc9 nuclear pore subcomplex via PKC--mediated phosphorylation of RanGAP1 Yujiao He1, Zhiguo Yang1†, Chen-si Zhao1†, Zhihui Xiao1†, Yu Gong1, Yun-Yi Li1, Yiqi Chen1, Yunting Du1, Dianying Feng1, Amnon Altman2, Yingqiu Li1* 1MOE Key Laboratory of Gene Function and Regulation, Guangdong Province Key Laboratory of Pharmaceutical Functional Genes, State Key Laboratory of Biocontrol, School of Life Sciences, Sun Yat-sen University, Guangzhou, China; 2Center for Cancer Immunotherapy, La Jolla Institute for Immunology, La Jolla, United States Abstract The nuclear pore complex (NPC) is the sole and selective gateway for nuclear transport, and its dysfunction has been associated with many diseases. The metazoan NPC subcomplex RanBP2, which consists of RanBP2 (Nup358), RanGAP1-SUMO1, and Ubc9, regulates the assembly and function of the NPC. The roles of immune signaling in regulation of NPC remain poorly understood. Here, we show that in human and murine T cells, following T-cell receptor (TCR) stimulation, protein kinase C-q (PKC-q) directly phosphorylates RanGAP1 to facilitate RanBP2 subcomplex assembly and nuclear import and, thus, the nuclear translocation of AP-1 transcription *For correspondence: factor. Mechanistically, TCR stimulation induces the translocation of activated PKC-q to the NPC, 504 506 [email protected] where it interacts with and phosphorylates RanGAP1 on Ser and Ser . RanGAP1 phosphorylation increases its binding affinity for Ubc9, thereby promoting sumoylation of RanGAP1 †These authors contributed and, finally, assembly of the RanBP2 subcomplex. Our findings reveal an unexpected role of PKC-q equally to this work as a direct regulator of nuclear import and uncover a phosphorylation-dependent sumoylation of Competing interests: The RanGAP1, delineating a novel link between TCR signaling and assembly of the RanBP2 NPC authors declare that no subcomplex. -
Supplementary Data
SUPPLEMENTARY DATA A cyclin D1-dependent transcriptional program predicts clinical outcome in mantle cell lymphoma Santiago Demajo et al. 1 SUPPLEMENTARY DATA INDEX Supplementary Methods p. 3 Supplementary References p. 8 Supplementary Tables (S1 to S5) p. 9 Supplementary Figures (S1 to S15) p. 17 2 SUPPLEMENTARY METHODS Western blot, immunoprecipitation, and qRT-PCR Western blot (WB) analysis was performed as previously described (1), using cyclin D1 (Santa Cruz Biotechnology, sc-753, RRID:AB_2070433) and tubulin (Sigma-Aldrich, T5168, RRID:AB_477579) antibodies. Co-immunoprecipitation assays were performed as described before (2), using cyclin D1 antibody (Santa Cruz Biotechnology, sc-8396, RRID:AB_627344) or control IgG (Santa Cruz Biotechnology, sc-2025, RRID:AB_737182) followed by protein G- magnetic beads (Invitrogen) incubation and elution with Glycine 100mM pH=2.5. Co-IP experiments were performed within five weeks after cell thawing. Cyclin D1 (Santa Cruz Biotechnology, sc-753), E2F4 (Bethyl, A302-134A, RRID:AB_1720353), FOXM1 (Santa Cruz Biotechnology, sc-502, RRID:AB_631523), and CBP (Santa Cruz Biotechnology, sc-7300, RRID:AB_626817) antibodies were used for WB detection. In figure 1A and supplementary figure S2A, the same blot was probed with cyclin D1 and tubulin antibodies by cutting the membrane. In figure 2H, cyclin D1 and CBP blots correspond to the same membrane while E2F4 and FOXM1 blots correspond to an independent membrane. Image acquisition was performed with ImageQuant LAS 4000 mini (GE Healthcare). Image processing and quantification were performed with Multi Gauge software (Fujifilm). For qRT-PCR analysis, cDNA was generated from 1 µg RNA with qScript cDNA Synthesis kit (Quantabio). qRT–PCR reaction was performed using SYBR green (Roche). -
SUPPLEMENTARY MATERIAL Bone Morphogenetic Protein 4 Promotes
www.intjdevbiol.com doi: 10.1387/ijdb.160040mk SUPPLEMENTARY MATERIAL corresponding to: Bone morphogenetic protein 4 promotes craniofacial neural crest induction from human pluripotent stem cells SUMIYO MIMURA, MIKA SUGA, KAORI OKADA, MASAKI KINEHARA, HIROKI NIKAWA and MIHO K. FURUE* *Address correspondence to: Miho Kusuda Furue. Laboratory of Stem Cell Cultures, National Institutes of Biomedical Innovation, Health and Nutrition, 7-6-8, Saito-Asagi, Ibaraki, Osaka 567-0085, Japan. Tel: 81-72-641-9819. Fax: 81-72-641-9812. E-mail: [email protected] Full text for this paper is available at: http://dx.doi.org/10.1387/ijdb.160040mk TABLE S1 PRIMER LIST FOR QRT-PCR Gene forward reverse AP2α AATTTCTCAACCGACAACATT ATCTGTTTTGTAGCCAGGAGC CDX2 CTGGAGCTGGAGAAGGAGTTTC ATTTTAACCTGCCTCTCAGAGAGC DLX1 AGTTTGCAGTTGCAGGCTTT CCCTGCTTCATCAGCTTCTT FOXD3 CAGCGGTTCGGCGGGAGG TGAGTGAGAGGTTGTGGCGGATG GAPDH CAAAGTTGTCATGGATGACC CCATGGAGAAGGCTGGGG MSX1 GGATCAGACTTCGGAGAGTGAACT GCCTTCCCTTTAACCCTCACA NANOG TGAACCTCAGCTACAAACAG TGGTGGTAGGAAGAGTAAAG OCT4 GACAGGGGGAGGGGAGGAGCTAGG CTTCCCTCCAACCAGTTGCCCCAAA PAX3 TTGCAATGGCCTCTCAC AGGGGAGAGCGCGTAATC PAX6 GTCCATCTTTGCTTGGGAAA TAGCCAGGTTGCGAAGAACT p75 TCATCCCTGTCTATTGCTCCA TGTTCTGCTTGCAGCTGTTC SOX9 AATGGAGCAGCGAAATCAAC CAGAGAGATTTAGCACACTGATC SOX10 GACCAGTACCCGCACCTG CGCTTGTCACTTTCGTTCAG Suppl. Fig. S1. Comparison of the gene expression profiles of the ES cells and the cells induced by NC and NC-B condition. Scatter plots compares the normalized expression of every gene on the array (refer to Table S3). The central line -
The E3 Ligase PIAS1 Regulates P53 Sumoylation to Control Stress-Induced Apoptosis of Lens
fcell-09-660494 June 14, 2021 Time: 13:5 # 1 ORIGINAL RESEARCH published: 14 June 2021 doi: 10.3389/fcell.2021.660494 The E3 Ligase PIAS1 Regulates p53 Sumoylation to Control Stress-Induced Apoptosis of Lens Edited by: Wolfgang Knabe, Epithelial Cells Through the Universität Münster, Germany Proapoptotic Regulator Bax Reviewed by: Guillaume Bossis, † † † † † Centre National de la Recherche Qian Nie , Huimin Chen , Ming Zou , Ling Wang , Min Hou , Jia-Wen Xiang, Scientifique (CNRS), France Zhongwen Luo, Xiao-Dong Gong, Jia-Ling Fu, Yan Wang, Shu-Yu Zheng, Yuan Xiao, Stefan Müller, Yu-Wen Gan, Qian Gao, Yue-Yue Bai, Jing-Miao Wang, Lan Zhang, Xiang-Cheng Tang, Goethe University Frankfurt, Germany Xuebin Hu, Lili Gong, Yizhi Liu* and David Wan-Cheng Li*‡ Leena Latonen, University of Eastern Finland, Finland State Key Laboratory of Ophthalmology, Zhongshan Ophthalmic Center, Sun Yat-sen University, Guangzhou, China *Correspondence: David Wan-Cheng Li Protein sumoylation is one of the most important post-translational modifications [email protected] Yizhi Liu regulating many biological processes (Flotho A & Melchior F. 2013. Ann Rev. Biochem. [email protected] 82:357–85). Our previous studies have shown that sumoylation plays a fundamental †These authors have contributed role in regulating lens differentiation (Yan et al., 2010. PNAS, 107(49):21034-9.; equally to this work Gong et al., 2014. PNAS. 111(15):5574–9). Whether sumoylation is implicated in lens ‡ ORCID: David Wan-Cheng Li pathogenesis remains elusive. Here, we present evidence to show that the protein orcid.org/0000-0002-7398-7630 inhibitor of activated STAT-1 (PIAS1), a E3 ligase for sumoylation, is implicated in regulating stress-induced lens pathogenesis.