Civil Engineering Systems and Their Evolution
Total Page:16
File Type:pdf, Size:1020Kb
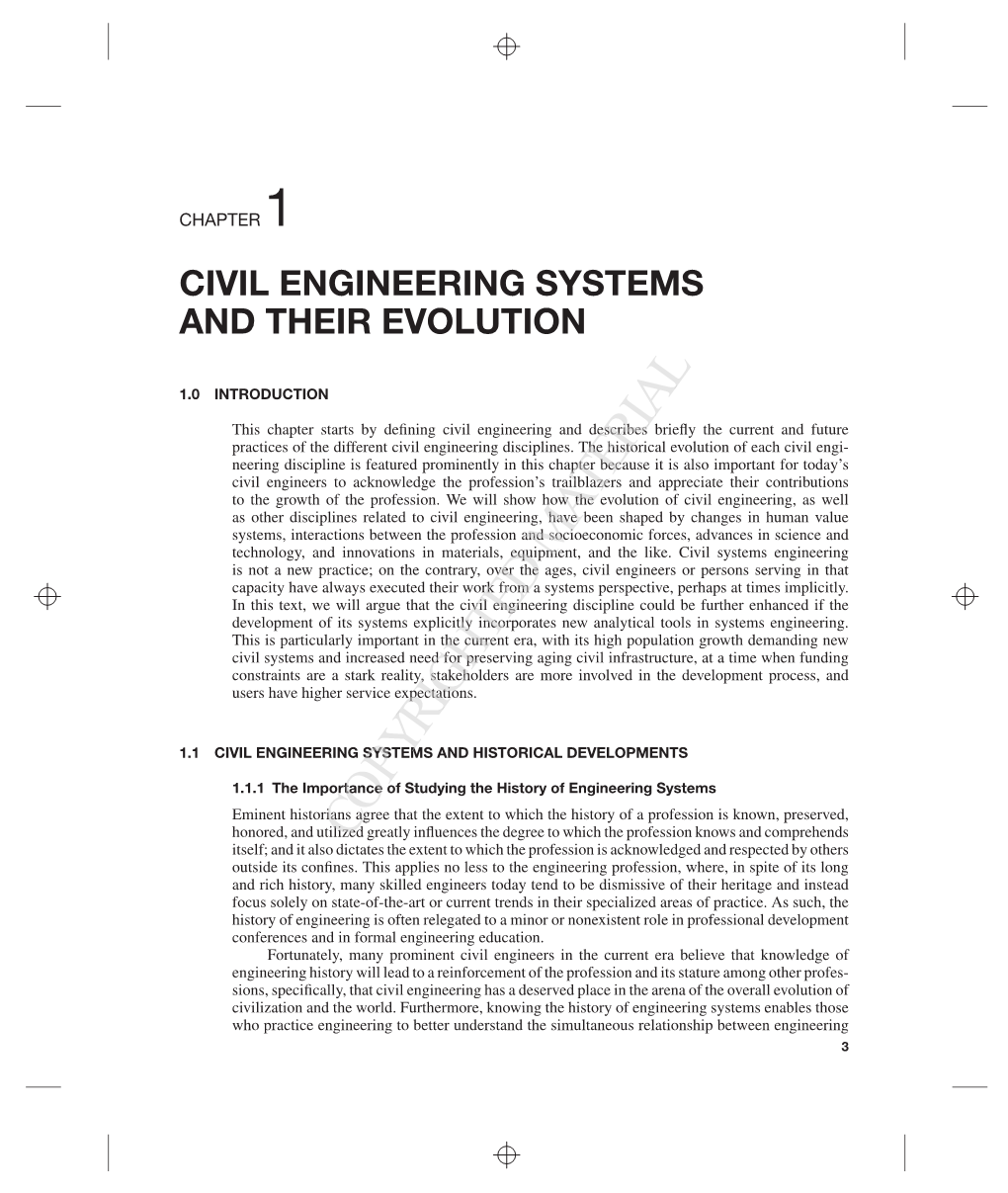
Load more
Recommended publications
-
Robotics/Principles of Technology (Semester Course)
Robotics/Principles of Technology (Semester Course) Course Description Robotics/Principles of Technology is aligned with the NGSS standards and was developed based upon the interest of students. Through the use of technology, engineering and robotics, the course stresses problem‐solving skills in a real world setting. Students will be given tasks and will need to design and construct various projects. Students will work independently as well as in pairs, small groups and large groups. Both verbal and written communication of ideas will be emphasized. The course is designed to motivate the student of all ability levels to participate in real‐world scenarios utilizing engineering and robotic concepts to problem solve through hand on activities. Robotics/Principles of Technology encompass STEM principles in a variety of in‐class and out‐of‐class learning activities designed to teach the student about careers in technology, engineering, robotics and science. Suggested Course Sequence: History of Robotics (2 weeks) Careers in STEM (1 week) Design in Engineering & Technology (2‐3 weeks) Getting Started in Robotics (3‐4 weeks) Space Exploration Robotics (6‐7 weeks) Design & Build a Robot (3 weeks) ENGAGING STUDENTS • FOSTERING ACHIEVEMENT • CULTIVATING 21st CENTURY GLOBAL SKILLS Unit Overview Content Area: Robotics/Principles of Technology Unit Title: History of Robots Grade Level: 11‐12 Unit Summary: This unit will cover the development of robotics over time. Students will research how robotics has evolved over time. Interdisciplinary Connections: History 6.1.4.C.17 6.1.4.C.16 English RT.11‐12.9 RT.11‐12.10 WHST.11‐12.2B Technology 8.1.12.E.1 8.2.12.A.3 8.2.12.C.2 21st Century Themes and Skills: ‐ CRP2 Apply appropriate academic and technical skills. -
IMPREGILO GROUP Origins
PROGRESS, OUR GREATEST WORK 2008 Annual Report 2008 The value of a group is tied to its history and concessions market, particularly for motorway IMPREGILO GROUP origins. networks, renewable energy plants and energy 2008 Annual Report Impregilo group was set up at the beginning of the transportation. It is also active in the plant and 1990s but its origins lie much further back as it is engineering and environmental services sectors. the legacy of Girola, Lodigini, Impresit and Cogefar. Dams, hydroelectric plants, motorways, railways, These prestigious Italian companies were at the underground train systems, tunnels, bridges, forefront of international civil engineering from the viaducts, desalination plants, fume treatment plants early twentieth century. and waste-to-energy plants: a wealth of experience gained in Italy and abroad thanks to its constant Active in all five continents, the companies that commitment to meeting deadlines, protecting the merged to become Impregilo engaged in the environment and deploying innovative technologies. IMPREGILO GROUP construction of the main motorway, railway and hydroelectric works underpinning development in Impregilo is a group looking to the future. It is Italy and in many other countries around the world, responsible and sensitive to its stakeholders’ and helped strengthen Italy’s international standing. expectations and has proved itself well capable of exploiting and anticipating market developments This rich history of tradition and success has made over its more than a hundred years of experience. Impregilo, listed on the Italian stock exchange, the leading general contractor in Italy and one of the Today, thanks to its business and organisational major international general construction companies. -
Nanotechnology, Ethics and Policy Education: Research and Pedagogy: Selected Bibliography
Association for Practical and Professional Ethics Conference Presentation, March 2013 NSF Nanotechnology Undergraduate Education grant (#EEC-1138257) focused on creating interdisciplinary modules about the social, ethical, environmental, and economic impact of nanotechnology for all students at the Colorado School of Mines. (For an overview of the project plan, see NanoSTEP: Nano-Science, Technology, Ethics and Policy Poster Presentation to NSF EEC conference March 2012 ) Dr. Corinne Packard, a professor in the department of Metallurgical and Materials Engineering who works with solar cell nanomaterials, was the PI. The team of Co-PIs and Senior Personnel were drawn from the multidisciplinary Liberal Arts and International Studies department which is responsible for delivering core courses in humanities and social sciences. The first part of the project was a module for the freshman ethics and writing course, Nature and Human Values, which included a common lecture and reading assignments and different course activates and discussions about the risks and benefits of the technology. (See Workshop and Module Design—Part 1—Nature and Human Values). The second part of the project was a module for the sophomore-level course, Human Systems, a history of sociological, religious, political, and economic systems. For this course, the focus of the module was on policy and international relations in technology development. (See Workshop and Module Design—Part 2—Human Systems). We disseminated our work at several conferences and to universities in China and Spain, but most notably contributed a panel discussion with several team members at APPE 2013 in San Antonio (See Association for Practical and Professional Ethics 2013 Conference Presentations). -
Interim Financial Report
(Translation from the Italian original which remains the definitive version) Interim financial report 30 June 2018 This document is available at: www.salini-impregilo.com Salini Impregilo S.p.A. Company managed and coordinated by Salini Costruttori S.p.A. Salini Impregilo S.p.A. Share capital €544,740,000 Registered office in Milan, Via dei Missaglia 97 Tax code and Milan Company Registration no. 00830660155 R.E.A. no. 525502 - VAT no. 02895590962 1 CONTENTS Company officers ................................................................................................................................................................... 3 Key events of the period......................................................................................................................................................... 4 Directors’ report - Part I ................................................................................................................. 5 Financial highlights ............................................................................................................................................................ 6 Performance ...................................................................................................................................................................... 9 Directors’ report - Part II .............................................................................................................. 20 Performance by geographical segment .......................................................................................................................... -
The Evolution of Engineering Materials
Paper ID #20611 The Evolution of Engineering Materials Dr. Amber L. Genau, University of Alabama at Birmingham Dr. Amber Genau is an assistant professor in the Materials Science and Engineering Department at the University of Alabama at Birmingham. She received her B.S. and M.S. from Iowa State University and Ph.D. from Northwestern University, all in materials engineering. Before coming to UAB, Dr. Genau spent two years as a guest scientist at the German Aerospace Center in Cologne, Germany, working on metal solidification and microstructural characterization. She is particularly interested in broadening participation in engineering and providing international experiences and perspectives to undergraduate students. c American Society for Engineering Education, 2017 The Evolution of Engineering Materials Abstract This paper describes the development of an upper level engineering elective entitled “The Evolution of Engineering Materials.” The course considers how the discovery of new materials and the ability of process materials in new ways has influenced the course of history, shaping both human societies and their surrounding environments, from the Stone Age to the Modern Era. Students become familiar with a variety of still-relevant technical content through the consideration of historical activity, from smelting and coking to polymerization reactions and cross linking. The course addresses a variety of ABET outcomes while also supporting the development of global competency through an increased appreciation of world history. Although this course was developed for and taught in the context of a three-week study abroad trip to Europe, the engaging and accessible nature of the content could also make it valuable as a service course for non-engineering majors. -
Chemical & Biological Engineering Department History (PDF)
Chemical & Biological Engineering The University of Alabama A Century of Excellence and Quality A Legacy of Leadership 1910 – 2010 By Gary C. April University Research Professor & Head (Emeritus) A Century of Excellence and Quality A Legacy of Leadership 1910-2010 Gary C. April University Research Professor & Head Emeritus Chemical & Biological Engineering The University of Alabama October 2010 ii Dedication The author would like to dedicate this brief history of the Chemical Engineering Department at The University of Alabama to Lynne, his wife, partner and soul mate of 48 years, to his children, Andrew, Brian and Elizabeth, who lived most of their lives while he was a professor there, and to his granddaughters, Andrea, Abigail, Caroline and Olivia who continue to provide him with encouragement by sharing a vision for the future that is filled with hope and opportunities. iii Table of Contents Preface ..................................................................................................................... v 1. The Beginning of Engineering Education at The University of Alabama 1 ................................................................................................................. 1 2. The Emergence of the Chemical Engineering Profession ........................... 7 3. Chemical Engineering at the University of Alabama – A Century of Excellence and Quality – A Legacy of Leadership 5 ....................................... 11 4. The Significance of Who We Are ................................................................. -
Annual Report 2017 Report 2017
ANNUAL ANNUAL REPORT 2017 REPORT 2017 I ANNUAL REPORT 31 DECEMBER 2017 TABLE OF CONTENTS CEO’S LETTER TO THE SHAREHOLDERS 4 COMPANY OFFICERS 12 OUR VISION AND GLOBAL PRESENCE 14 KEY EVENTS OF THE YEAR 16 DIRECTORS’ REPORT - PART I 22 FINANCIAL HIGHLIGHTS 24 PERFORMANCE 32 DIRECTORS’ REPORT - PART II 54 PERFORMANCE BY GEOGRAPHICAL SEGMENT 58 RISK MANAGEMENT SYSTEM 88 MAIN RISK FACTORS AND UNCERTAINTIES 96 EVENTS AFTER THE REPORTING DATE 126 OUTLOOK 128 REPORT ON CORPORATE GOVERNANCE AND THE OWNERSHIP STRUCTURE 130 ALTERNATIVE PERFORMANCE INDICATORS 132 OTHER INFORMATION 136 2017 CONSOLIDATED NON-FINANCIAL STATEMENT 138 CONSOLIDATED FINANCIAL STATEMENTS AS AT AND FOR THE YEAR ENDED 31 DECEMBER 2017 210 NOTES TO THE CONSOLIDATED FINANCIAL STATEMENTS 220 Statement of financial position 266 Statement of profit or loss 367 CONSOLIDATED FINANCIAL STATEMENTS OF SALINI IMPREGILO GROUP - INTRAGROUP TRANSACTIONS 388 CONSOLIDATED FINANCIAL STATEMENTS OF SALINI IMPREGILO GROUP - EQUITY INVESTMENTS 404 CONSOLIDATED FINANCIAL STATEMENTS OF SALINI IMPREGILO GROUP - LIST OF COMPANIES 416 STATEMENT ON THE CONSOLIDATED FINANCIAL STATEMENTS 438 SEPARATE FINANCIAL STATEMENTS OF SALINI IMPREGILO S.P.A. AS AT AND FOR THE YEAR ENDED 31 DECEMBER 2017 440 NOTES TO THE SEPARATE FINANCIAL STATEMENTS 450 Statement of financial position 482 Statement of profit or loss 545 Proposal to the shareholders of Salini Impregilo S.p.A. 566 SEPARATE FINANCIAL STATEMENTS OF SALINI IMPREGILO - INTRAGROUP TRANSACTIONS 568 SEPARATE FINANCIAL STATEMENTS OF SALINI IMPREGILO - EQUITY INVESTMENTS 588 STATEMENT ON THE SEPARATE FINANCIAL STATEMENTS 608 REPORTS 610 CEO’S LETTER TO THE SHAREHOLDERS Directors’ Report 6 ANNUAL REPORT 2017 Dear Shareholders, effective as shown not just by the numbers and the attained results, but also by the market’s acknowledgement. -
Displacement and Equilibrium: a Cultural History of Engineering in America Before Its “Golden Age”
Displacement and Equilibrium: A Cultural History of Engineering in America Before Its “Golden Age” A DISSERTATION SUBMITTED TO THE FACULTY OF UNIVERSITY OF MINNESOTA BY David M. Kmiec IN PARTIAL FULFILLMENT OF THE REQUIREMENTS FOR THE DEGREE OF DOCTOR OF PHILOSOPHY Bernadette Longo, Adviser August 2012 Table of Contents Introduction ............................................................................................................. 1 1 Using historical context to contextualize history: The ideology of cultural history ........................................................................... 12 Motivation and methodology for a cultural history of American engineering ......................................................................................... 16 2 National service and public work: Military education and civilian engineering ......................................................... 23 Early modern France and the origins of military engineering ................................ 24 Military engineering and the American Revolution ................................................ 30 Civilian engineering as rationale for maintaining a military in peacetime .............................................................................................. 37 Engineering union in sectionalist America .............................................................. 51 3 The self-made engineer goes to school: Institutionalized education(s) for engineers ......................................................... 59 Colleges at the -
History of Engineering and Development of Industrial Engineering
History of Engineering and Development of Industrial Engineering Department of Industrial Engineering Çankaya University Fall’2015 References • http://www.youtube.com/watch?v=nGx73g3mbEA • http://www.youtube.com/watch?v=3K3-stVK0lM • http://www.introtoie.com/ • http://www.iienet2.org/details.aspx?id=716 • ... 2 Science and Engineering – Early Developments • How did the two words industrial and engineering become combined to form the label industrial engineering? • relationship of industrial engineering to other engineering disciplines • To understand the role of industrial engineering (IE) in today’s complex world, it is helpful to learn the historical developments involved in the progress of IE. • Science is concerned with the search for basic knowledge. • Engineering is concerned with the application of scientific knowledge. • Engineering and science have been developed in a parallel, complementary fashion, although not always at the same pace. 3 Science and Engineering – Early Developments • Engineering provides feedback to science in areas where new knowledge is needed. • In earlier times, although science and engineering each have different characteristics / are different disciplines, they are the same person • Leonardo di ser Piero da Vinci (April 15, 1452 – May 2, 1519) was an Italian scientist, mathematician, engineer, inventor, anatomist, painter, sculptor, architect, botanist, musician, writer. • The person who discovered the knowledge also put into use • The Pyramids • the Great Wall of China • The Roman Construction Projects • Efforts to provide a better life 4 Science and Engineering – Early Developments • Almost all engineering developments prior to 1800 had to do with physical phenomena: • Overcoming friction • Lifting • Construction • Later developments were concerned with chemical and molecular phenomena: • Electricty • Properties of materials • Thermal processes • Combustion • Other chemical processes 5 Science and Engineering – Early Developments • Fundemental to almost all engineering developments were the advances made in mathematics. -
Italy-9-Index.Pdf
© Lonely Planet 925 Index A Alpe di Fanes 339-41 Brescia 285-7 AbbaziaABBREVIATIONS di Pomposa 463-4 Alpe di Siusi 338 Cagliari 839 AbbaziaACT di SanAustralian Galgano 544Capital Alta Badia 339, 340 Catania 795 Territory Abbazia di Sant’Antimo 550 Alta Murgia National Park 85 Catanzaro 750 NSW New South Wales Abruzzo 619-29, 622 alte vie hiking trails 316 Cuma 658-9 NT Northern Territory Abruzzo Lazio e Molise National Altipiano della Paganella 322-4 Fiesole 511 Qld Queensland Park 85 Alto Adige 313-16, 329-42, 318-19 Filicudi 786 SA South Australia abseiling 800, 866 Alto Lario 305 Herculaneum 671-2 Tas Tasmania AC Milan 276 Altopiano del Golgo 869-70 itineraries 32 Vic Victoria Accademia Carrara 283 Amalfi 685-7, 686 Lecce 723-4 WA Western Australia accommodation 871-5 Amalfi Coast 87, 681-91, 12 Lipari 780 agriturismo 21, 22, 579, 872 Ampezzo 424 Metaponto 733 B&Bs 872 amusement parks Naples 643 camping 698, 872-3 Aquafàn 471 Nora 844 convents 873 Aquaparadise 309 nuraghi 851, 852, 857, 859, 863, farmstays 579 CanevaWorld 309 865, 867, 868 hostels 873 Delfinario Rimini 471 Ostia Antica 179 hotels 873-4 Fiabilandia 471 Paestum 691-2, 11 internet resources 874-5 Gardaland 309 Perugia 569 language 907 Italia in Miniatura 471 Pietrabbondante 632 monasteries 873 Movieland 309 Pompeii 674-5 mountain huts 874 Anacapri 663-4, 664 Pozzuoli 657-8 pensioni 873-4 Ancona 601-5, 602 Rimini 470 rental accommodation 874 Andalo 322 Saepinum 630-1 villa rentals 874-5 animals 81-3, see also individual Selinunte 822 Acquafredda di Maratea 740 species -
A History of American Engineering Education for Women Amy Bix Iowa State University, [email protected]
History Books History 1-2014 Girls Coming to Tech: A History of American Engineering Education for Women Amy Bix Iowa State University, [email protected] Follow this and additional works at: http://lib.dr.iastate.edu/history_books Part of the Engineering Education Commons, and the Women's History Commons Recommended Citation Bix, Amy, "Girls Coming to Tech: A History of American Engineering Education for Women" (2014). History Books. 4. http://lib.dr.iastate.edu/history_books/4 This Book is brought to you for free and open access by the History at Iowa State University Digital Repository. It has been accepted for inclusion in History Books by an authorized administrator of Iowa State University Digital Repository. For more information, please contact [email protected]. CONTENTS SERIES FOREWORD IX ACKNOWLEDGMENTS XI INTRODUCTION RARE INVADERS: THE PRE-WORLD WAR II HISTORY OF WOMEN IN AMERICAN ENGINEERING 29 2 WORLD WAR II: EMERGENCY ENGINEERING EMPLOYMENT TRAINING 55 3 NEW WARTIME AND POSIWAR ENGINEERING MAJORS: PURDUE, RPI. COLUMBIA 95 4 COEDUCATION VIA LAWSUIT: GEORGIA TECH I 3 I 5 COEDUCATION FOR SOCIAL LIFE: CALTECH I 9 I 6 A SPECIAL CASE: WOMEN AT MIT 223 7 CHANGING THE CLIMATE 255 NOTES 299 INDEX 353 WLEDGMENTS .1cJimsey, as INTRODUCTION nee in help my mistakes >ssible with ler scholarly :he National nia Institute : University, rersity, Uni :y ofMichi versity. I am •ant to offer Engineering education in the United States has a gendered history that until relatively a State Uni recently prevented women from finding a comfortable place in the predominantly on multiple male technical world. Throughout the nineteenth century and most of the twentieth, American observers treated the professional study of technology as men's territory. -
Orthotropic Steel Deck Bridges in the U.S
ORTHOTROPIC STEEL DECK BRIDGES IN THE U.S. Brian M. Kozy1 and Justin Ocel2 Abstract This paper summarizes some of the recent developments in the U.S. on the subject of orthotropic steel deck (OSD) bridges. The Federal Highway Administration published a manual to provide up-to-date technical guidance on the proper design, construction and maintenance of OSDs for bridges and the AASHTO bridge design specifications have been greatly revised and expanded. The rib-to-deck weld continues to be an area of difficulty in OSD construction; due to competing desire for fabrication economy and fatigue longevity in the detail. Preliminary results from FHWA research on this detail are presented. Background Many of the world’s notable major bridge structures utilize the orthotropic steel plate system as one of the basic structural building blocks for distribution of traffic loads in decks and for the stiffening of slender plate elements in compression. Examples include the new San Francisco Oakland Bay Bridge, Self-Anchored Suspension Span in California and the proposed Strait of Messina Bridge in Italy. Stiffened steel plates have been used for many years in a wide range of steel construction applications. They are particularly prevalent in the ship building industry and for hydraulic applications such as tanks, gates, and locks. The first orthotropic steel deck (OSD) bridge was developed by German engineers in the 1930's and the first such deck was constructed in 1936. In the United States, a similar system was built and often referred to as a “battle deck” because it was considered to be as strong as a battleship.