Discovery and Characterization of Three of These Transiting Circumbinary Planetary Systems, Specifically Kepler-47, Kepler-64, Kepler-413
Total Page:16
File Type:pdf, Size:1020Kb
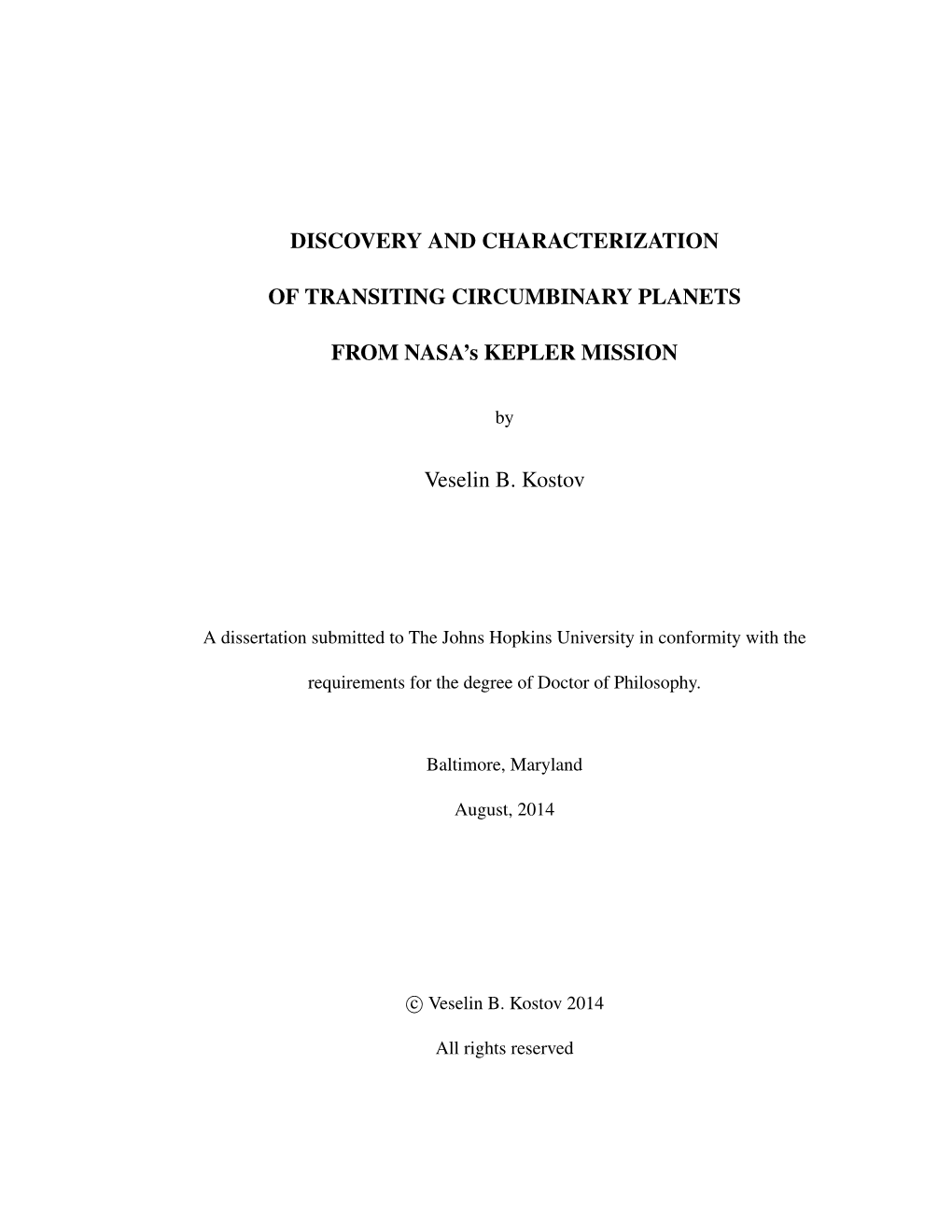
Load more
Recommended publications
-
Lurking in the Shadows: Wide-Separation Gas Giants As Tracers of Planet Formation
Lurking in the Shadows: Wide-Separation Gas Giants as Tracers of Planet Formation Thesis by Marta Levesque Bryan In Partial Fulfillment of the Requirements for the Degree of Doctor of Philosophy CALIFORNIA INSTITUTE OF TECHNOLOGY Pasadena, California 2018 Defended May 1, 2018 ii © 2018 Marta Levesque Bryan ORCID: [0000-0002-6076-5967] All rights reserved iii ACKNOWLEDGEMENTS First and foremost I would like to thank Heather Knutson, who I had the great privilege of working with as my thesis advisor. Her encouragement, guidance, and perspective helped me navigate many a challenging problem, and my conversations with her were a consistent source of positivity and learning throughout my time at Caltech. I leave graduate school a better scientist and person for having her as a role model. Heather fostered a wonderfully positive and supportive environment for her students, giving us the space to explore and grow - I could not have asked for a better advisor or research experience. I would also like to thank Konstantin Batygin for enthusiastic and illuminating discussions that always left me more excited to explore the result at hand. Thank you as well to Dimitri Mawet for providing both expertise and contagious optimism for some of my latest direct imaging endeavors. Thank you to the rest of my thesis committee, namely Geoff Blake, Evan Kirby, and Chuck Steidel for their support, helpful conversations, and insightful questions. I am grateful to have had the opportunity to collaborate with Brendan Bowler. His talk at Caltech my second year of graduate school introduced me to an unexpected population of massive wide-separation planetary-mass companions, and lead to a long-running collaboration from which several of my thesis projects were born. -
Naming the Extrasolar Planets
Naming the extrasolar planets W. Lyra Max Planck Institute for Astronomy, K¨onigstuhl 17, 69177, Heidelberg, Germany [email protected] Abstract and OGLE-TR-182 b, which does not help educators convey the message that these planets are quite similar to Jupiter. Extrasolar planets are not named and are referred to only In stark contrast, the sentence“planet Apollo is a gas giant by their assigned scientific designation. The reason given like Jupiter” is heavily - yet invisibly - coated with Coper- by the IAU to not name the planets is that it is consid- nicanism. ered impractical as planets are expected to be common. I One reason given by the IAU for not considering naming advance some reasons as to why this logic is flawed, and sug- the extrasolar planets is that it is a task deemed impractical. gest names for the 403 extrasolar planet candidates known One source is quoted as having said “if planets are found to as of Oct 2009. The names follow a scheme of association occur very frequently in the Universe, a system of individual with the constellation that the host star pertains to, and names for planets might well rapidly be found equally im- therefore are mostly drawn from Roman-Greek mythology. practicable as it is for stars, as planet discoveries progress.” Other mythologies may also be used given that a suitable 1. This leads to a second argument. It is indeed impractical association is established. to name all stars. But some stars are named nonetheless. In fact, all other classes of astronomical bodies are named. -
The Minor Planet Bulletin
THE MINOR PLANET BULLETIN OF THE MINOR PLANETS SECTION OF THE BULLETIN ASSOCIATION OF LUNAR AND PLANETARY OBSERVERS VOLUME 36, NUMBER 3, A.D. 2009 JULY-SEPTEMBER 77. PHOTOMETRIC MEASUREMENTS OF 343 OSTARA Our data can be obtained from http://www.uwec.edu/physics/ AND OTHER ASTEROIDS AT HOBBS OBSERVATORY asteroid/. Lyle Ford, George Stecher, Kayla Lorenzen, and Cole Cook Acknowledgements Department of Physics and Astronomy University of Wisconsin-Eau Claire We thank the Theodore Dunham Fund for Astrophysics, the Eau Claire, WI 54702-4004 National Science Foundation (award number 0519006), the [email protected] University of Wisconsin-Eau Claire Office of Research and Sponsored Programs, and the University of Wisconsin-Eau Claire (Received: 2009 Feb 11) Blugold Fellow and McNair programs for financial support. References We observed 343 Ostara on 2008 October 4 and obtained R and V standard magnitudes. The period was Binzel, R.P. (1987). “A Photoelectric Survey of 130 Asteroids”, found to be significantly greater than the previously Icarus 72, 135-208. reported value of 6.42 hours. Measurements of 2660 Wasserman and (17010) 1999 CQ72 made on 2008 Stecher, G.J., Ford, L.A., and Elbert, J.D. (1999). “Equipping a March 25 are also reported. 0.6 Meter Alt-Azimuth Telescope for Photometry”, IAPPP Comm, 76, 68-74. We made R band and V band photometric measurements of 343 Warner, B.D. (2006). A Practical Guide to Lightcurve Photometry Ostara on 2008 October 4 using the 0.6 m “Air Force” Telescope and Analysis. Springer, New York, NY. located at Hobbs Observatory (MPC code 750) near Fall Creek, Wisconsin. -
Livre-Ovni.Pdf
UN MONDE BIZARRE Le livre des étranges Objets Volants Non Identifiés Chapitre 1 Paranormal Le paranormal est un terme utilisé pour qualifier un en- mé n'est pas considéré comme paranormal par les semble de phénomènes dont les causes ou mécanismes neuroscientifiques) ; ne sont apparemment pas explicables par des lois scien- tifiques établies. Le préfixe « para » désignant quelque • Les différents moyens de communication avec les chose qui est à côté de la norme, la norme étant ici le morts : naturels (médiumnité, nécromancie) ou ar- consensus scientifique d'une époque. Un phénomène est tificiels (la transcommunication instrumentale telle qualifié de paranormal lorsqu'il ne semble pas pouvoir que les voix électroniques); être expliqué par les lois naturelles connues, laissant ain- si le champ libre à de nouvelles recherches empiriques, à • Les apparitions de l'au-delà (fantômes, revenants, des interprétations, à des suppositions et à l'imaginaire. ectoplasmes, poltergeists, etc.) ; Les initiateurs de la parapsychologie se sont donné comme objectif d'étudier d'une manière scientifique • la cryptozoologie (qui étudie l'existence d'espèce in- ce qu'ils considèrent comme des perceptions extra- connues) : classification assez injuste, car l'objet de sensorielles et de la psychokinèse. Malgré l'existence de la cryptozoologie est moins de cultiver les mythes laboratoires de parapsychologie dans certaines universi- que de chercher s’il y a ou non une espèce animale tés, notamment en Grande-Bretagne, le paranormal est inconnue réelle derrière une légende ; généralement considéré comme un sujet d'étude peu sé- rieux. Il est en revanche parfois associé a des activités • Le phénomène ovni et ses dérivés (cercle de culture). -
Henryk Siemiradzki and the International Artistic Milieu
ACCADEMIA POL ACCA DELLE SCIENZE DELLE SCIENZE POL ACCA ACCADEMIA BIBLIOTECA E CENTRO DI STUDI A ROMA E CENTRO BIBLIOTECA ACCADEMIA POLACCA DELLE SCIENZE BIBLIOTECA E CENTRO DI STUDI A ROMA CONFERENZE 145 HENRYK SIEMIRADZKI AND THE INTERNATIONAL ARTISTIC MILIEU FRANCESCO TOMMASINI, L’ITALIA E LA RINASCITA E LA RINASCITA L’ITALIA TOMMASINI, FRANCESCO IN ROME DELLA INDIPENDENTE POLONIA A CURA DI MARIA NITKA AGNIESZKA KLUCZEWSKA-WÓJCIK CONFERENZE 145 ACCADEMIA POLACCA DELLE SCIENZE BIBLIOTECA E CENTRO DI STUDI A ROMA ISSN 0239-8605 ROMA 2020 ISBN 978-83-956575-5-9 CONFERENZE 145 HENRYK SIEMIRADZKI AND THE INTERNATIONAL ARTISTIC MILIEU IN ROME ACCADEMIA POLACCA DELLE SCIENZE BIBLIOTECA E CENTRO DI STUDI A ROMA CONFERENZE 145 HENRYK SIEMIRADZKI AND THE INTERNATIONAL ARTISTIC MILIEU IN ROME A CURA DI MARIA NITKA AGNIESZKA KLUCZEWSKA-WÓJCIK. ROMA 2020 Pubblicato da AccademiaPolacca delle Scienze Bibliotecae Centro di Studi aRoma vicolo Doria, 2 (Palazzo Doria) 00187 Roma tel. +39 066792170 e-mail: [email protected] www.rzym.pan.pl Il convegno ideato dal Polish Institute of World Art Studies (Polski Instytut Studiów nad Sztuką Świata) nell’ambito del programma del Ministero della Scienza e dell’Istruzione Superiore della Repubblica di Polonia (Polish Ministry of Science and Higher Education) “Narodowy Program Rozwoju Humanistyki” (National Programme for the Develop- ment of Humanities) - “Henryk Siemiradzki: Catalogue Raisonné of the Paintings” (“Tradition 1 a”, no. 0504/ nprh4/h1a/83/2015). Il convegno è stato organizzato con il supporto ed il contributo del National Institute of Polish Cultural Heritage POLONIKA (Narodowy Instytut Polskiego Dziedzictwa Kul- turowego za Granicą POLONIKA). Redazione: Maria Nitka, Agnieszka Kluczewska-Wójcik Recensione: Prof. -
Fy10 Budget by Program
AURA/NOAO FISCAL YEAR ANNUAL REPORT FY 2010 Revised Submitted to the National Science Foundation March 16, 2011 This image, aimed toward the southern celestial pole atop the CTIO Blanco 4-m telescope, shows the Large and Small Magellanic Clouds, the Milky Way (Carinae Region) and the Coal Sack (dark area, close to the Southern Crux). The 33 “written” on the Schmidt Telescope dome using a green laser pointer during the two-minute exposure commemorates the rescue effort of 33 miners trapped for 69 days almost 700 m underground in the San Jose mine in northern Chile. The image was taken while the rescue was in progress on 13 October 2010, at 3:30 am Chilean Daylight Saving time. Image Credit: Arturo Gomez/CTIO/NOAO/AURA/NSF National Optical Astronomy Observatory Fiscal Year Annual Report for FY 2010 Revised (October 1, 2009 – September 30, 2010) Submitted to the National Science Foundation Pursuant to Cooperative Support Agreement No. AST-0950945 March 16, 2011 Table of Contents MISSION SYNOPSIS ............................................................................................................ IV 1 EXECUTIVE SUMMARY ................................................................................................ 1 2 NOAO ACCOMPLISHMENTS ....................................................................................... 2 2.1 Achievements ..................................................................................................... 2 2.2 Status of Vision and Goals ................................................................................ -
A Basic Requirement for Studying the Heavens Is Determining Where In
Abasic requirement for studying the heavens is determining where in the sky things are. To specify sky positions, astronomers have developed several coordinate systems. Each uses a coordinate grid projected on to the celestial sphere, in analogy to the geographic coordinate system used on the surface of the Earth. The coordinate systems differ only in their choice of the fundamental plane, which divides the sky into two equal hemispheres along a great circle (the fundamental plane of the geographic system is the Earth's equator) . Each coordinate system is named for its choice of fundamental plane. The equatorial coordinate system is probably the most widely used celestial coordinate system. It is also the one most closely related to the geographic coordinate system, because they use the same fun damental plane and the same poles. The projection of the Earth's equator onto the celestial sphere is called the celestial equator. Similarly, projecting the geographic poles on to the celest ial sphere defines the north and south celestial poles. However, there is an important difference between the equatorial and geographic coordinate systems: the geographic system is fixed to the Earth; it rotates as the Earth does . The equatorial system is fixed to the stars, so it appears to rotate across the sky with the stars, but of course it's really the Earth rotating under the fixed sky. The latitudinal (latitude-like) angle of the equatorial system is called declination (Dec for short) . It measures the angle of an object above or below the celestial equator. The longitud inal angle is called the right ascension (RA for short). -
A Review on Substellar Objects Below the Deuterium Burning Mass Limit: Planets, Brown Dwarfs Or What?
geosciences Review A Review on Substellar Objects below the Deuterium Burning Mass Limit: Planets, Brown Dwarfs or What? José A. Caballero Centro de Astrobiología (CSIC-INTA), ESAC, Camino Bajo del Castillo s/n, E-28692 Villanueva de la Cañada, Madrid, Spain; [email protected] Received: 23 August 2018; Accepted: 10 September 2018; Published: 28 September 2018 Abstract: “Free-floating, non-deuterium-burning, substellar objects” are isolated bodies of a few Jupiter masses found in very young open clusters and associations, nearby young moving groups, and in the immediate vicinity of the Sun. They are neither brown dwarfs nor planets. In this paper, their nomenclature, history of discovery, sites of detection, formation mechanisms, and future directions of research are reviewed. Most free-floating, non-deuterium-burning, substellar objects share the same formation mechanism as low-mass stars and brown dwarfs, but there are still a few caveats, such as the value of the opacity mass limit, the minimum mass at which an isolated body can form via turbulent fragmentation from a cloud. The least massive free-floating substellar objects found to date have masses of about 0.004 Msol, but current and future surveys should aim at breaking this record. For that, we may need LSST, Euclid and WFIRST. Keywords: planetary systems; stars: brown dwarfs; stars: low mass; galaxy: solar neighborhood; galaxy: open clusters and associations 1. Introduction I can’t answer why (I’m not a gangstar) But I can tell you how (I’m not a flam star) We were born upside-down (I’m a star’s star) Born the wrong way ’round (I’m not a white star) I’m a blackstar, I’m not a gangstar I’m a blackstar, I’m a blackstar I’m not a pornstar, I’m not a wandering star I’m a blackstar, I’m a blackstar Blackstar, F (2016), David Bowie The tenth star of George van Biesbroeck’s catalogue of high, common, proper motion companions, vB 10, was from the end of the Second World War to the early 1980s, and had an entry on the least massive star known [1–3]. -
Solar Writer Report for Abraham Lincoln
FIXED STARS A Solar Writer Report for Abraham Lincoln Written by Diana K Rosenberg Compliments of:- Stephanie Johnson Seeing With Stars Astrology PO Box 159 Stepney SA 5069 Australia Tel/Fax: +61 (08) 8331 3057 Email: [email protected] Web: www.esotech.com.au Page 2 Abraham Lincoln Natal Chart 12 Feb 1809 12:40:56 PM UT +0:00 near Hodgenville 37°N35' 085°W45' Tropical Placidus 22' 13° 08°ˆ ‡ 17' ¾ 06' À ¿É ‰ 03° ¼ 09° 00° 06° 09°06° ˆ ˆ ‡ † ‡ 25° 16' 41'08' 40' † 01' 09' Œ 29' ‰ 9 10 23° ¶ 8 27°‰ 11 Ï 27° 01' ‘ ‰02' á 7 12 ‘ áá 23° á 23° ¸ 23°Š27' á Š à „ 28' 28' 6 18' 1 10°‹ º ‹37' 13° 05' ‹ 5 Á 22° ½ 27' 2 4 01' Ü 3 07° Œ ƒ » 09' 23° 09° Ý Ü 06° 16' 06' Ê 00°ƒ 13° 22' Ý 17' 08°‚ Page 23 Astrological Summary Chart Point Positions: Abraham Lincoln Planet Sign Position House Comment The Moon Capricorn 27°Cp01' 12th The Sun Aquarius 23°Aq27' 12th read into 1st House Mercury Pisces 10°Pi18' 1st Venus Aries 7°Ar27' 1st read into 2nd House Mars Libra 25°Li29' 8th Jupiter Pisces 22°Pi05' 1st Saturn Sagittarius 3°Sg08' 9th read into 10th House Uranus Scorpio 9°Sc40' 8th Neptune Sagittarius 6°Sg41' 9th read into 10th House Pluto Pisces 13°Pi37' 1st The North Node Scorpio 6°Sc09' 8th The South Node Taurus 6°Ta09' 2nd The Ascendant Aquarius 23°Aq28' 1st The Midheaven Sagittarius 8°Sg22' 10th The Part of Fortune Capricorn 27°Cp02' 12th Chart Point Aspects Planet Aspect Planet Orb App/Sep The Moon Square Mars 1°32' Separating The Moon Conjunction The Part of Fortune 0°00' Applying The Sun Trine Mars 2°02' Applying The Sun Conjunction The Ascendant -
16Th HEAD Meeting Session Table of Contents
16th HEAD Meeting Sun Valley, Idaho – August, 2017 Meeting Abstracts Session Table of Contents 99 – Public Talk - Revealing the Hidden, High Energy Sun, 204 – Mid-Career Prize Talk - X-ray Winds from Black Rachel Osten Holes, Jon Miller 100 – Solar/Stellar Compact I 205 – ISM & Galaxies 101 – AGN in Dwarf Galaxies 206 – First Results from NICER: X-ray Astrophysics from 102 – High-Energy and Multiwavelength Polarimetry: the International Space Station Current Status and New Frontiers 300 – Black Holes Across the Mass Spectrum 103 – Missions & Instruments Poster Session 301 – The Future of Spectral-Timing of Compact Objects 104 – First Results from NICER: X-ray Astrophysics from 302 – Synergies with the Millihertz Gravitational Wave the International Space Station Poster Session Universe 105 – Galaxy Clusters and Cosmology Poster Session 303 – Dissertation Prize Talk - Stellar Death by Black 106 – AGN Poster Session Hole: How Tidal Disruption Events Unveil the High 107 – ISM & Galaxies Poster Session Energy Universe, Eric Coughlin 108 – Stellar Compact Poster Session 304 – Missions & Instruments 109 – Black Holes, Neutron Stars and ULX Sources Poster 305 – SNR/GRB/Gravitational Waves Session 306 – Cosmic Ray Feedback: From Supernova Remnants 110 – Supernovae and Particle Acceleration Poster Session to Galaxy Clusters 111 – Electromagnetic & Gravitational Transients Poster 307 – Diagnosing Astrophysics of Collisional Plasmas - A Session Joint HEAD/LAD Session 112 – Physics of Hot Plasmas Poster Session 400 – Solar/Stellar Compact II 113 -
Astronomy with Small Telescopes
Astronomy With Small Telescopes Bohdan Paczy´nski Princeton University Observatory, Princeton, NJ 08544 [email protected] ABSTRACT The All Sky Automated Survey (ASAS) is monitoring all sky to about 14 mag with a cadence of about 1 day; it has discovered about 105 variable stars, most of them new. The instrument used for the survey had aperture of 7 cm. A search for planetary transits has lead to the discovery of about a dozen confirmed planets, so called ’hot Jupiters’, providing the information of planetary masses and radii. Most discoveries were done with telescopes with aperture of 10 cm. We propose a search for optical transients covering all sky with a cadence of 10 - 30 minutes and the limit of 12 - 14 mag, with an instant verification of all candidate events. The search will be made with a large number of 10 cm instruments, and the verification will be done with 30 cm instruments. We also propose a system to be located at the L1 point of the Earth - Sun system to detect ’killer asteroids’. With a limiting magnitude of about 18 mag it could detect 10 m boulders several hours prior to their impact, provide warning against Tunguska-like events, as well as to provide news about spectacular but harmless more modest impacts. Subject headings: techniques: photometric — surveys — celestial mechanics — mete- oroids — stars: variable — gamma rays: bursts arXiv:astro-ph/0609161v3 7 Nov 2006 1. Introduction The goal of this paper is to point out that there are many tasks for which small and even very small telescopes are not only useful, but even indispensable. -
IAU Division C Working Group on Star Names 2019 Annual Report
IAU Division C Working Group on Star Names 2019 Annual Report Eric Mamajek (chair, USA) WG Members: Juan Antonio Belmote Avilés (Spain), Sze-leung Cheung (Thailand), Beatriz García (Argentina), Steven Gullberg (USA), Duane Hamacher (Australia), Susanne M. Hoffmann (Germany), Alejandro López (Argentina), Javier Mejuto (Honduras), Thierry Montmerle (France), Jay Pasachoff (USA), Ian Ridpath (UK), Clive Ruggles (UK), B.S. Shylaja (India), Robert van Gent (Netherlands), Hitoshi Yamaoka (Japan) WG Associates: Danielle Adams (USA), Yunli Shi (China), Doris Vickers (Austria) WGSN Website: https://www.iau.org/science/scientific_bodies/working_groups/280/ WGSN Email: [email protected] The Working Group on Star Names (WGSN) consists of an international group of astronomers with expertise in stellar astronomy, astronomical history, and cultural astronomy who research and catalog proper names for stars for use by the international astronomical community, and also to aid the recognition and preservation of intangible astronomical heritage. The Terms of Reference and membership for WG Star Names (WGSN) are provided at the IAU website: https://www.iau.org/science/scientific_bodies/working_groups/280/. WGSN was re-proposed to Division C and was approved in April 2019 as a functional WG whose scope extends beyond the normal 3-year cycle of IAU working groups. The WGSN was specifically called out on p. 22 of IAU Strategic Plan 2020-2030: “The IAU serves as the internationally recognised authority for assigning designations to celestial bodies and their surface features. To do so, the IAU has a number of Working Groups on various topics, most notably on the nomenclature of small bodies in the Solar System and planetary systems under Division F and on Star Names under Division C.” WGSN continues its long term activity of researching cultural astronomy literature for star names, and researching etymologies with the goal of adding this information to the WGSN’s online materials.