Track 3: Mission Concepts and Policy for Nuclear Space Systems
Total Page:16
File Type:pdf, Size:1020Kb
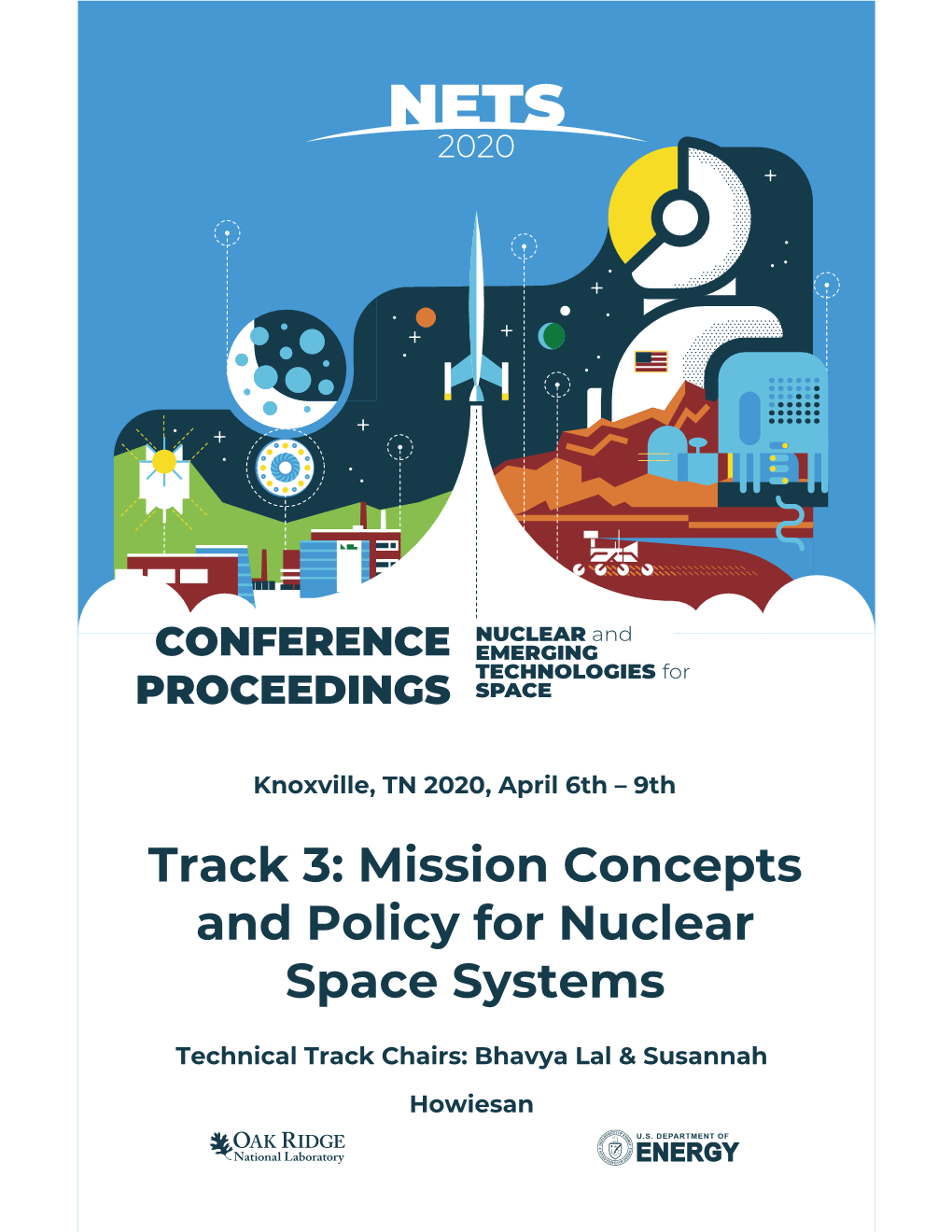
Load more
Recommended publications
-
Mission to Jupiter
This book attempts to convey the creativity, Project A History of the Galileo Jupiter: To Mission The Galileo mission to Jupiter explored leadership, and vision that were necessary for the an exciting new frontier, had a major impact mission’s success. It is a book about dedicated people on planetary science, and provided invaluable and their scientific and engineering achievements. lessons for the design of spacecraft. This The Galileo mission faced many significant problems. mission amassed so many scientific firsts and Some of the most brilliant accomplishments and key discoveries that it can truly be called one of “work-arounds” of the Galileo staff occurred the most impressive feats of exploration of the precisely when these challenges arose. Throughout 20th century. In the words of John Casani, the the mission, engineers and scientists found ways to original project manager of the mission, “Galileo keep the spacecraft operational from a distance of was a way of demonstrating . just what U.S. nearly half a billion miles, enabling one of the most technology was capable of doing.” An engineer impressive voyages of scientific discovery. on the Galileo team expressed more personal * * * * * sentiments when she said, “I had never been a Michael Meltzer is an environmental part of something with such great scope . To scientist who has been writing about science know that the whole world was watching and and technology for nearly 30 years. His books hoping with us that this would work. We were and articles have investigated topics that include doing something for all mankind.” designing solar houses, preventing pollution in When Galileo lifted off from Kennedy electroplating shops, catching salmon with sonar and Space Center on 18 October 1989, it began an radar, and developing a sensor for examining Space interplanetary voyage that took it to Venus, to Michael Meltzer Michael Shuttle engines. -
Distinguished Property in Tensor Products and Weak* Dual Spaces
axioms Article Distinguished Property in Tensor Products and Weak* Dual Spaces Salvador López-Alfonso 1 , Manuel López-Pellicer 2,* and Santiago Moll-López 3 1 Department of Architectural Constructions, Universitat Politècnica de València, 46022 Valencia, Spain; [email protected] 2 Emeritus and IUMPA, Universitat Politècnica de València, 46022 Valencia, Spain 3 Department of Applied Mathematics, Universitat Politècnica de València, 46022 Valencia, Spain; [email protected] * Correspondence: [email protected] 0 Abstract: A local convex space E is said to be distinguished if its strong dual Eb has the topology 0 0 0 0 b(E , (Eb) ), i.e., if Eb is barrelled. The distinguished property of the local convex space Cp(X) of real- valued functions on a Tychonoff space X, equipped with the pointwise topology on X, has recently aroused great interest among analysts and Cp-theorists, obtaining very interesting properties and nice characterizations. For instance, it has recently been obtained that a space Cp(X) is distinguished if and only if any function f 2 RX belongs to the pointwise closure of a pointwise bounded set in C(X). The extensively studied distinguished properties in the injective tensor products Cp(X) ⊗# E and in Cp(X, E) contrasts with the few distinguished properties of injective tensor products related to the dual space Lp(X) of Cp(X) endowed with the weak* topology, as well as to the weak* dual of Cp(X, E). To partially fill this gap, some distinguished properties in the injective tensor product space Lp(X) ⊗# E are presented and a characterization of the distinguished property of the weak* dual of Cp(X, E) for wide classes of spaces X and E is provided. -
Space) Barriers for 50 Years: the Past, Present, and Future of the Dod Space Test Program
SSC17-X-02 Breaking (Space) Barriers for 50 Years: The Past, Present, and Future of the DoD Space Test Program Barbara Manganis Braun, Sam Myers Sims, James McLeroy The Aerospace Corporation 2155 Louisiana Blvd NE, Suite 5000, Albuquerque, NM 87110-5425; 505-846-8413 [email protected] Colonel Ben Brining USAF SMC/ADS 3548 Aberdeen Ave SE, Kirtland AFB NM 87117-5776; 505-846-8812 [email protected] ABSTRACT 2017 marks the 50th anniversary of the Department of Defense Space Test Program’s (STP) first launch. STP’s predecessor, the Space Experiments Support Program (SESP), launched its first mission in June of 1967; it used a Thor Burner II to launch an Army and a Navy satellite carrying geodesy and aurora experiments. The SESP was renamed to the Space Test Program in July 1971, and has flown over 568 experiments on over 251 missions to date. Today the STP is managed under the Air Force’s Space and Missile Systems Center (SMC) Advanced Systems and Development Directorate (SMC/AD), and continues to provide access to space for DoD-sponsored research and development missions. It relies heavily on small satellites, small launch vehicles, and innovative approaches to space access to perform its mission. INTRODUCTION Today STP continues to provide access to space for DoD-sponsored research and development missions, Since space first became a viable theater of operations relying heavily on small satellites, small launch for the Department of Defense (DoD), space technologies have developed at a rapid rate. Yet while vehicles, and innovative approaches to space access. -
Mars 2020 Radiological Contingency Planning
National Aeronautics and Space Administration Mars 2020 Radiological Contingency Planning NASA plans to launch the Mars 2020 rover, produce the rover’s onboard power and to Perseverance, in summer 2020 on a mission warm its internal systems during the frigid to seek signs of habitable conditions in Mars’ Martian night. ancient past and search for signs of past microbial life. The mission will lift off from Cape NASA prepares contingency response plans Canaveral Air Force Station in Florida aboard a for every launch that it conducts. Ensuring the United Launch Alliance Atlas V launch vehicle safety of launch-site workers and the public in between mid-July and August 2020. the communities surrounding the launch area is the primary consideration in this planning. The Mars 2020 rover design is based on NASA’s Curiosity rover, which landed on Mars in 2012 This contingency planning task takes on an and greatly increased our knowledge of the added dimension when the payload being Red Planet. The Mars 2020 rover is equipped launched into space contains nuclear material. to study its landing site in detail and collect and The primary goal of radiological contingency store the most promising samples of rock and planning is to enable an efficient response in soil on the surface of Mars. the event of an accident. This planning is based on the fundamental principles of advance The system that provides electrical power for preparation (including rehearsals of simulated Mars 2020 and its scientific equipment is the launch accident responses), the timely availability same as for the Curiosity rover: a Multi- of technically accurate and reliable information, Mission Radioisotope Thermoelectric Generator and prompt external communication with the (MMRTG). -
MULTIPHYSICS DESIGN and SIMULATION of a TUNGSTEN-CERMET NUCLEAR THERMAL ROCKET a Thesis by BRAD APPEL Submitted to the Office O
MULTIPHYSICS DESIGN AND SIMULATION OF A TUNGSTEN-CERMET NUCLEAR THERMAL ROCKET A Thesis by BRAD APPEL Submitted to the Office of Graduate Studies of Texas A&M University in partial fulfillment of the requirements for the degree of MASTER OF SCIENCE August 2012 Major Subject: Nuclear Engineering Multiphysics Design and Simulation of a Tungsten-Cermet Nuclear Thermal Rocket Copyright 2012 Brad Appel ii MULTIPHYSICS DESIGN AND SIMULATION OF A TUNGSTEN-CERMET NUCLEAR THERMAL ROCKET A Thesis by BRAD APPEL Submitted to the Office of Graduate Studies of Texas A&M University in partial fulfillment of the requirements for the degree of MASTER OF SCIENCE Approved by: Chair of Committee, Karen Vierow Committee Members, Shannon Bragg-Sitton Paul Cizmas Head of Department, Yassin Hassan August 2012 Major Subject: Nuclear Engineering iii iii ABSTRACT Multiphysics Design and Simulation of a Tungsten-Cermet Nuclear Thermal Rocket. (August 2012) Brad Appel, B.S., Purdue University Chair of Advisory Committee: Dr. Karen Vierow The goal of this research is to apply modern methods of analysis to the design of a tungsten-cermet Nuclear Thermal Rocket (NTR) core. An NTR is one of the most viable propulsion options for enabling piloted deep-space exploration. Concerns over fuel safety have sparked interest in an NTR core based on tungsten-cermet fuel. This work investigates the capability of modern CFD and neutronics codes to design a cermet NTR, and makes specific recommendations for the configuration of channels in the core. First, the best CFD practices available from the commercial package Star-CCM+ are determined by comparing different modeling options with a hot-hydrogen flow experiment. -
NETS 2020 Template
بÀƵƧǘȁǞƧƊǶ §ȲȌǐȲƊǿ ƊƧDzɈȌɈǘƵwȌȌȁƊȁƮȌȁ ɈȌwƊȲȺɈǘȲȌɐǐǘƊƮɨƊȁƧǞȁǐ خȁɐƧǶƵƊȲɈƵƧǘȁȌǶȌǐǞƵȺƊȁƮ ǞȁȁȌɨƊɈǞȌȁ ǞȺ ȺȯȌȁȺȌȲƵƮ Ʀɯ ɈǘƵ ƊDz ªǞƮǐƵ yƊɈǞȌȁƊǶ ׁׂ׀ׂ y0À² ÀǘǞȺ ƧȌȁǏƵȲƵȁƧƵ خׁׂ׀ׂ ةɈǘ׀׃ƊȁƮ ɩǞǶǶƦƵ ǘƵǶƮ ǏȲȌǿȯȲǞǶ ׂ׆ɈǘٌةmƊƦȌȲƊɈȌȲɯ ɩǞǶǶ ƦƵ ǘƵǶƮ ɨǞȲɈɐƊǶǶɯ ȺȌ ɈǘƊɈ ɈǘƵ ƵȁɈǞȲƵ y0À² خƧȌǿǿɐȁǞɈɯǿƊɯȯƊȲɈǞƧǞȯƊɈƵǞȁɈǘǞȺƵɮƧǞɈǞȁǐǿƵƵɈǞȁǐ ǐȌɨخȌȲȁǶخخׁׂ׀ȁƵɈȺׂششبǘɈɈȯȺ Nuclear and Emerging Technologies for Space Sponsored by Oak Ridge National Laboratory, April 26th-30th, 2021. Available online at https://nets2021.ornl.gov Table of Contents Table of Contents .................................................................................................................................................... 1 Thanks to the NETS2021 Sponsors! ...................................................................................................................... 2 Nuclear and Emerging Technologies for Space 2021 – Schedule at a Glance ................................................. 3 Nuclear and Emerging Technologies for Space 2021 – Technical Sessions and Panels By Track ............... 6 Nuclear and Emerging Technologies for Space 2021 – Lightning Talk Final Program ................................... 8 Nuclear and Emerging Technologies for Space 2021 – Track 1 Final Program ............................................. 11 Nuclear and Emerging Technologies for Space 2021 – Track 2 Final Program ............................................. 14 Nuclear and Emerging Technologies for Space 2021 – Track 3 Final Program ............................................. 18 -
MARS 2020, CANDIDATES LANDING SITES, EXOMARS and THEIR RELATIONSHIP to MARS SAMPLE RETURN 10:25 A.M
2nd International Mars Sample Return 2018 (LPI Contrib. No. 2071) sess303.pdf Thursday, April 26, 2018 MARS 2020, CANDIDATES LANDING SITES, EXOMARS AND THEIR RELATIONSHIP TO MARS SAMPLE RETURN 10:25 a.m. Forum This session will present a status report on the Mars 2020 sample-caching rover, its candidate landing sites, the Exomars rover mission, and the relationship of all of these to Mars Sample Return. Chairs: Victoria Hipkin (Canadian Space Agency, Canada) Briony Horgan (Purdue University, USA) 10:25 a.m. Williford K. * *Jet Propulsion Laboratory, California Institute of Technology, USA The NASA Mars 2020 Rover Mission The NASA Mars 2020 rover mission will explore an astrobiologically relevant site to characterize its geology, evaluate past habitability, seek signs of ancient life, and assemble a returnable cache of samples. An overview of technical capabilities and scientific strategy in development will be presented with a focus on the role envisioned for Mars 2020 in a possible Mars sample return campaign. 10:45 a.m. Schulte M. * Meyer M. Grant J. Golombek M. *NASA Headquarters, USA The Mars 2020 Rover Mission Landing Site Candidates [#6026] The number of suitable landing sites for the Mars 2020 rover mission has been narrowed to three leading candidates: Jezero Crater, NE Syrtis, and Columbia Hills. Each offers geologic settings with the potential for preservation of biosignatures. 11:00 a.m. Vago J. L. * Svedhem H. Sefton-Nash E. Kminek G. Ruesch O. Haldemann A. F. C. Rodionov D. ExoMars Science Working Team *European Space Agency ExoMars Contributions to Mars Sample Return [#6021] Each of the two ExoMars missions has the potential to make discoveries that could inform and perhaps influence our collective strategy for a future Mars Sample Return mission. -
Lightweight, High-Temperature Radiator for In-Space Nuclear- Electric Power and Propulsion
University of Massachusetts Amherst ScholarWorks@UMass Amherst Doctoral Dissertations Dissertations and Theses Summer November 2014 Lightweight, High-Temperature Radiator for In-Space Nuclear- Electric Power and Propulsion Briana N. Tomboulian University of Massachusetts Amherst Follow this and additional works at: https://scholarworks.umass.edu/dissertations_2 Part of the Heat Transfer, Combustion Commons, Propulsion and Power Commons, and the Systems Engineering and Multidisciplinary Design Optimization Commons Recommended Citation Tomboulian, Briana N., "Lightweight, High-Temperature Radiator for In-Space Nuclear-Electric Power and Propulsion" (2014). Doctoral Dissertations. 247. https://doi.org/10.7275/5972048.0 https://scholarworks.umass.edu/dissertations_2/247 This Open Access Dissertation is brought to you for free and open access by the Dissertations and Theses at ScholarWorks@UMass Amherst. It has been accepted for inclusion in Doctoral Dissertations by an authorized administrator of ScholarWorks@UMass Amherst. For more information, please contact [email protected]. Lightweight, High-Temperature Radiator for In-Space Nuclear-Electric Power and Propulsion A Dissertation Presented by BRIANA N. TOMBOULIAN Submitted to the Graduate School of the University of Massachusetts Amherst in partial fulfillment of the requirements for the degree of DOCTOR OF PHILOSOPHY September 2014 Mechanical and Industrial Engineering Department © Copyright by Briana N. Tomboulian 2014 All Rights Reserved Lightweight, High-Temperature Radiator -
Inventory of CO2 Available for Terraforming Mars
PERSPECTIVE https://doi.org/10.1038/s41550-018-0529-6 Inventory of CO2 available for terraforming Mars Bruce M. Jakosky 1,2* and Christopher S. Edwards3 We revisit the idea of ‘terraforming’ Mars — changing its environment to be more Earth-like in a way that would allow terres- trial life (possibly including humans) to survive without the need for life-support systems — in the context of what we know about Mars today. We want to answer the question of whether it is possible to mobilize gases present on Mars today in non- atmospheric reservoirs by emplacing them into the atmosphere, and increase the pressure and temperature so that plants or humans could survive at the surface. We ask whether this can be achieved considering realistic estimates of available volatiles, without the use of new technology that is well beyond today’s capability. Recent observations have been made of the loss of Mars’s atmosphere to space by the Mars Atmosphere and Volatile Evolution Mission probe and the Mars Express space- craft, along with analyses of the abundance of carbon-bearing minerals and the occurrence of CO2 in polar ice from the Mars Reconnaissance Orbiter and the Mars Odyssey spacecraft. These results suggest that there is not enough CO2 remaining on Mars to provide significant greenhouse warming were the gas to be emplaced into the atmosphere; in addition, most of the CO2 gas in these reservoirs is not accessible and thus cannot be readily mobilized. As a result, we conclude that terraforming Mars is not possible using present-day technology. he concept of terraforming Mars has been a mainstay of sci- Could the remaining planetary inventories of CO2 be mobi- ence fiction for a long time, but it also has been discussed from lized and emplaced into the atmosphere via current or plausible 1 a scientific perspective, initially by Sagan and more recently near-future technologies? Would the amount of CO2 that could T 2 by, for example, McKay et al. -
Mars Reconnaissance Orbiter Maneuver Plan for Mars 2020 Entry, Descent, and Landing Support and Beyond
Jet Propulsion Laboratory California Ins5tute of Technology Mars Reconnaissance Orbiter Maneuver Plan for Mars 2020 Entry, Descent, and Landing Support and Beyond Sean Wagner Premkumar Menon AAS/AIAA Space Flight Mechanics Meeting Ka'anapali, HI January 13{17, 2019 AAS Paper 19-233 c 2019 California Institute of Technology. U.S. Government sponsorship acknowledged. Jet Propulsion Laboratory MarsCalifornia Ins5tute of Technology Reconnaissance Orbiter Project Mars Reconnaissance Orbiter Mission The Mars Reconnaissance Orbiter launched on August 12, 2005 from the Cape Canaveral Air Force Station and arrived at Mars on March 10, 2006. After aerobraking, MRO began the Primary Science Phase (PSP) in November 2006 and continues to perform science observations at Mars. MRO Spacecraft: Spacecraft Bus: 3-axis stabilized ACS system; 3-meter diameter High Gain Antenna; hydrazine propulsion system Instrument Suite: HiRISE Camera, CRISM Imaging Spectrometer, Mars Climate Sounder, Mars Color Imager, Context Camera, Shallow Subsurface Radar, Electra Proximity Link Payload (among other instrument payloads) January 15, 2019 MRO Maneuver Plan for Mars 2020 EDL Support and Beyond SVW { 3 MRO Primary Science Orbit (PSO) The MRO PSO is designed to satisfy science and mission requirements; the spacecraft is flown in an orbit designed to optimize the science instruments performance. The MRO PSO is defined by three key characteristics: Sun-synchronous orbit ascending node at 3:00 PM ± 15 minutes Local Mean Solar Time (LMST) (daylight equatorial crossing) Periapsis -
Espinsights the Global Space Activity Monitor
ESPInsights The Global Space Activity Monitor Issue 2 May–June 2019 CONTENTS FOCUS ..................................................................................................................... 1 European industrial leadership at stake ............................................................................ 1 SPACE POLICY AND PROGRAMMES .................................................................................... 2 EUROPE ................................................................................................................. 2 9th EU-ESA Space Council .......................................................................................... 2 Europe’s Martian ambitions take shape ......................................................................... 2 ESA’s advancements on Planetary Defence Systems ........................................................... 2 ESA prepares for rescuing Humans on Moon .................................................................... 3 ESA’s private partnerships ......................................................................................... 3 ESA’s international cooperation with Japan .................................................................... 3 New EU Parliament, new EU European Space Policy? ......................................................... 3 France reflects on its competitiveness and defence posture in space ...................................... 3 Germany joins consortium to support a European reusable rocket......................................... -
Power Systems Design
Power Systems Design • Lecture #17 – October 27, 2020 • Definitions of energy and power • Power generation systems • Energy storage systems • Integrated systems analysis © 2020 David L. Akin - All rights reserved http://spacecraft.ssl.umd.edu U N I V E R S I T Y O F Space Power Systems Design ENAE 483/788D - Principles of Space Systems Design MARYLAND 1 Energy and Power - Not the Same!!! • Energy - the capacity of a physical system to do work (J, N-m, kWhr) • Power - time rate of change of energy (W, N-m/ sec, J/sec) • We are interested in generating power; we store and use energy at a given power level. U N I V E R S I T Y O F Space Power Systems Design ENAE 483/788D - Principles of Space Systems Design MARYLAND 2 Solar Power • Insolation constant = 1394 W/m2 at 1 AU • Varies with inverse square of distance • Power conversion technologies – Photovoltaic – Thermodynamic cycle U N I V E R S I T Y O F Space Power Systems Design ENAE 483/788D - Principles of Space Systems Design MARYLAND 3 Solar Flux at Mars From Mason, Kilopower: Small Fission Power Systems for Mars and Beyond NASA FISO Working Group, Feb. 1, 2017 U N I V E R S I T Y O F Space Power Systems Design ENAE 483/788D - Principles of Space Systems Design MARYLAND 4 Photovoltaic Cell Efficiency U N I V E R S I T Y O F Space Power Systems Design ENAE 483/788D - Principles of Space Systems Design MARYLAND 5 Triple-Junction Photovoltaic Cell U N I V E R S I T Y O F Space Power Systems Design ENAE 483/788D - Principles of Space Systems Design MARYLAND 6 Individual Cell Efficiencies From Wertz, Everett, and Puschell, Space Mission Engineering: The New SMAD Microcosm Press, 2011 U N I V E R S I T Y O F Space Power Systems Design ENAE 483/788D - Principles of Space Systems Design MARYLAND 7 Photovoltaic Array Examples From Fortescue, Swinerd, and Stark, Spacecraft Systems Engineering, 4th ed.