Exchange and Fractionation of Mg Isotopes Between Epsomite and Saturated Mgso4 Solution
Total Page:16
File Type:pdf, Size:1020Kb
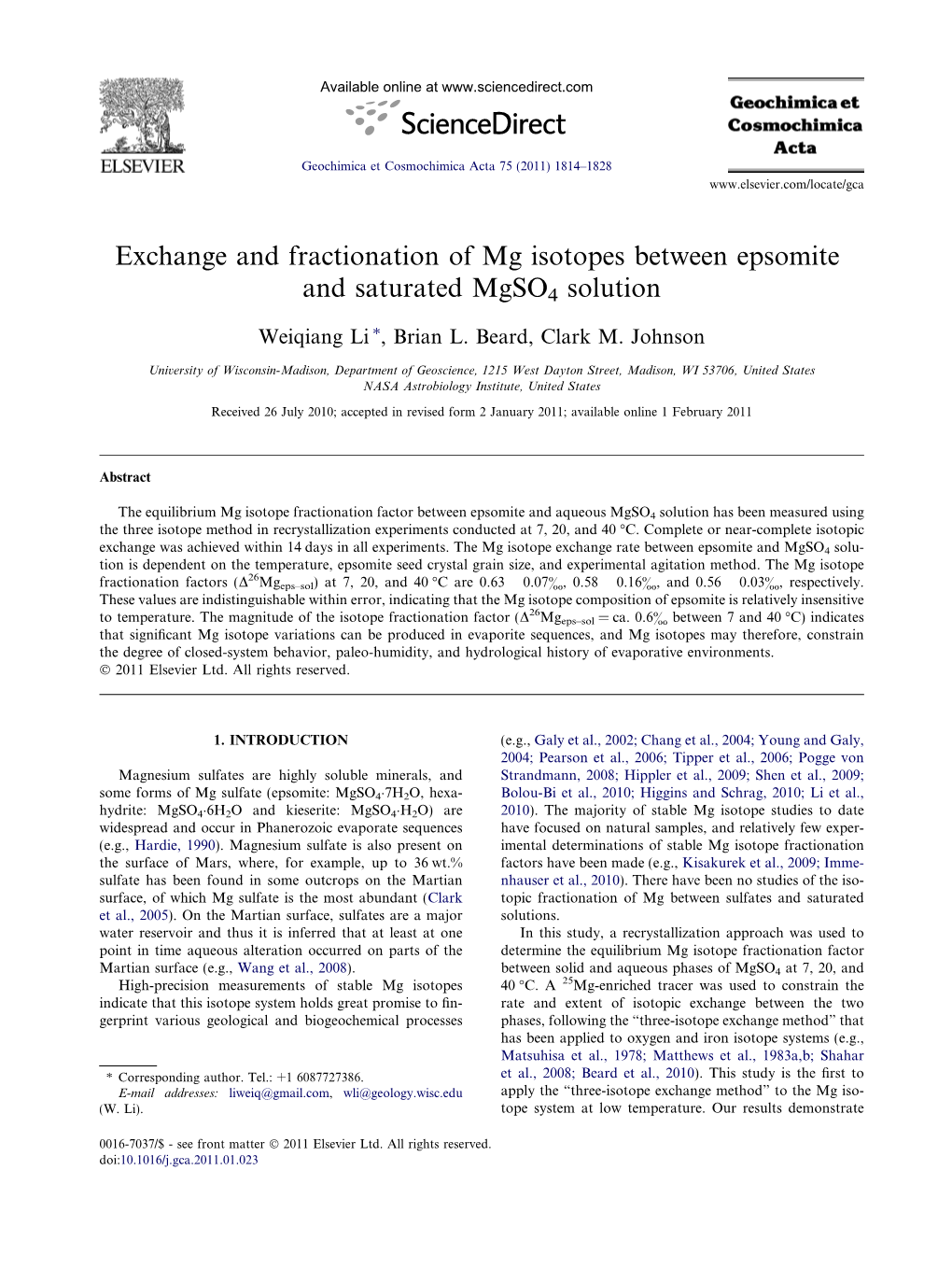
Load more
Recommended publications
-
Download PDF About Minerals Sorted by Mineral Name
MINERALS SORTED BY NAME Here is an alphabetical list of minerals discussed on this site. More information on and photographs of these minerals in Kentucky is available in the book “Rocks and Minerals of Kentucky” (Anderson, 1994). APATITE Crystal system: hexagonal. Fracture: conchoidal. Color: red, brown, white. Hardness: 5.0. Luster: opaque or semitransparent. Specific gravity: 3.1. Apatite, also called cellophane, occurs in peridotites in eastern and western Kentucky. A microcrystalline variety of collophane found in northern Woodford County is dark reddish brown, porous, and occurs in phosphatic beds, lenses, and nodules in the Tanglewood Member of the Lexington Limestone. Some fossils in the Tanglewood Member are coated with phosphate. Beds are generally very thin, but occasionally several feet thick. The Woodford County phosphate beds were mined during the early 1900s near Wallace, Ky. BARITE Crystal system: orthorhombic. Cleavage: often in groups of platy or tabular crystals. Color: usually white, but may be light shades of blue, brown, yellow, or red. Hardness: 3.0 to 3.5. Streak: white. Luster: vitreous to pearly. Specific gravity: 4.5. Tenacity: brittle. Uses: in heavy muds in oil-well drilling, to increase brilliance in the glass-making industry, as filler for paper, cosmetics, textiles, linoleum, rubber goods, paints. Barite generally occurs in a white massive variety (often appearing earthy when weathered), although some clear to bluish, bladed barite crystals have been observed in several vein deposits in central Kentucky, and commonly occurs as a solid solution series with celestite where barium and strontium can substitute for each other. Various nodular zones have been observed in Silurian–Devonian rocks in east-central Kentucky. -
Growth of Srb4o7 Crystal Fibers Along the C-Axis by Micro-Pulling-Down Method
crystals Article Growth of SrB4O7 Crystal Fibers along the c-Axis by Micro-Pulling-Down Method Ryouta Ishibashi, Harutoshi Asakawa * and Ryuichi Komatsu Graduate School of Science and Engineering, Yamaguchi University, Yamaguchi 753-8511, Japan; [email protected] (R.I.); [email protected] (R.K.) * Correspondence: [email protected]; Tel.: +81-836-85-9631 Abstract: SrB4O7 (SBO) receives much attention as solid-state ultraviolet lasers for micro-machining, photochemical synthesis, and laser spectroscopy. For the application of SBO, the SBO crystals require the control of twinning to amplify the conversion light. We also expected that the inhibitation of the SrB2O4 appearance was essential. Here, we show the growth of SBO crystals along the c-axis through the micro-pulling-down method while alternating the application of electric fields (E). Without the application, single crystals were grown. At E = 400 V/cm no needle domains of SrB2O4 inside SBO crystals existed; however, composition planes were formed and twin boundaries did not appear. In contrast, the inversion of surface morphology emerged, and the convex size was especially large at 1000 V/cm. These results demonstrate that convection is generated perpendicular to the growth front by alternating the application of electric fields. This surface morphological change contradicts the conventional concept of growth through the micro-pulling-down method. The distance from seed crystals vs. grain density plot also showed that the density did not decrease with a sufficient slope. Consequently, we concluded that the selection of the c-axis as growth faces is not fruitful to fabricate Citation: Ishibashi, R.; Asakawa, H.; twins, and the selection of the growth condition, under which geometrical selection strongly affects, Komatsu, R. -
Development Team
Material science Paper No. : Crystallography & crystal growth Module : Growth from melt II Development Team Prof. Vinay Gupta, Department of Physics and Astrophysics, Principal Investigator University of Delhi, Delhi Prof. P. N. Kotru ,Department of Physics, University of Jammu, Paper Coordinator Jammu-180006 Content Writer Prof. P. N. Kotru ,Department of Physics, University of Jammu, Jammu-180006 Prof Mahavir Singh Department of Physics, Himachal Pradesh Content Reviewer University, Shimla 1 Crystallography & crystal growth Material science Growth from melt II Description of Module Subject Name Physics Paper Name Crystallography & crystal growth Module Name/Title Growth from melt II Module Id 31 2 Crystallography & crystal growth Material science Growth from melt II 31 Bridgman-Stockbarger Growth Technique. 31.1 Introduction The techniques were originated by Bridgman (1925) and Stockbarger (1938) and so are named after them. In these techniques a crucible containing the material to be grown is lowered through a furnace in such a way that the lowest point in the crucible and the solidification surface rises slowly up the crucible. It means that the melt contained in the crucible is progressively frozen to yield a single crystal. The rate of lowering the crucible may range from about 0.1 to 200 mmh─1 but in most of the cases it may range somewhere in between 1-30 mmh─1. There are situations where the movement of the crucible is reversed. In other words, the crucible is raised up through the furnace and so is advantageously applicable for materials which are volatile; the interface with the vapour being the coolest part of the charge. -
Project Title: Embedded Nanocrystal Silicon Films: a New Paradigm for Improving the Stability of Thin-Film Silicon
Suite 1000 ME 111 Church St. SE Minneapolis, MN 55455-0111 Project Title: Embedded Nanocrystal Silicon Films: A New Paradigm for Improving the Stability of Thin-film Silicon Contract Number: RD-3-25 Report Date: 16 Dec 2011 Principal Investigator: Uwe Kortshagen Contract Contact: Amy Rollinger 612-625-4028 612-625-1359 Congressional District: (Corporate office) Minnesota 5th Congressional District: (Project location) Minnesota 5th FINAL REPORT Executive Summary: Under this grant, we pursued two different routes that may help increase the efficiency and lower the cost of thin film silicon solar cells. Our first approach (Track 1) is based on our unique ability to produce silicon nanocrystals in a low-pressure plasma-based synthesis reactor and to embed these nanocrystals in amorphous silicon films. Our novel deposition process enables us to independently control the properties of the amorphous matrix and of the crystalline phase, which we hope will enable us to improve the electronic quality of amorphous silicon that is used in thin film solar cells. In the second approach (Track 2), we study using such embedded nanocrystals as nuclei for seed-induced re-crystallization of amorphous silicon films. We expected that controlling the seed concentration will enable us to grow microcrystalline Si films faster and with grain sizes larger than possible with other deposition approaches. This may enable the cheaper production of solar cells based on microcrystalline silicon. The goals and objectives of this grant were achieved. This research led to two major conclusions: 1. The inclusion of Si nanocrystals into amorphous silicon leads to a mixed phase material that is more resistive to light-induced defect creation than standard amorphous silicon. -
Synthetic Quartz Crystal
Synthetic Quartz Crystal n Terms and Definitions Synthetic Quartz Crystal: A single crystal grown using the Right-handed and left-handed quartz crystals: Crystals are hydrothermal synthesis method. divided into two types: right-handed and left-handed. A As-Grown Quartz Crystal: A synthetic quartz crystal grown difference in optical rotation creates the 2 types, but their naturally with no processing. physical properties are identical. Therefore, by cutting at the Lumbered Quartz Crystal: A synthetic quartz crystal with the X correct angle, the difference does not affect the characteristics and Z surfaces processed according to specified dimensions of a crystal oscillator. Generally right-handed quartz crystals are and angles using a diamond wheel #80. used in manufacture. Y-bar Synthetic Quartz Crystal: A synthetic quartz crystal grown Zone: A zone with a crystal that has grown from a seed crystal at by using a bar-like seed crystal elongated in the Y-axis direction. its core. There are Z, +X, -X, and S zones. Z-plate Synthetic Quartz Crystal: A synthetic quartz crystal Infrared Absorption Coefficient α: This value measured with an grown by using a plate-like seed crystal with a Y-axis direction infrared spectrophotometer is adopted as the infrared absorption length and X-axis direction width. coefficient α of a synthetic quartz crystal. The value is based on Inclusion: A general term for solid constituents (inclusions) that the absorption characteristic of the OH radical of a synthetic exist in synthetic quartz crystal; they can be observed when light quartz crystal that is around 3,800 to 3,000 cm–1 of the infrared is scattered through a liquid with a refractive index that is close transmittance curve. -
On the Nature and Significance of Rarity in Mineralogy
1 1 REVISION #2—American Mineralogist—January 12, 2016 2 3 On the nature and significance of rarity in mineralogy 4 5 Robert M. Hazen1* and Jesse H. Ausubel2 6 1Geophysical Laboratory, Carnegie Institution, 5251 Broad Branch Road NW, Washington, D. C. 20015, USA. 7 2Program for the Human Environment, Rockefeller University, 1230 York Ave., New York, New York 10021, USA. 8 9 ABSTRACT 10 More than half of the >5000 approved mineral species are known from 5 or fewer localities 11 and thus are rare. Mineralogical rarity arises from different circumstances, but all rare mineral 12 species conform to one or more of 4 criteria: (1) P-T-X range: minerals that form only under 13 highly restricted conditions in pressure-temperature-composition space; (2) Planetary constraints: 14 minerals that incorporate essential elements that are rare or that form at extreme conditions that 15 seldom occur in Earth’s near-surface environment; (3) Ephemeral phases: minerals that rapidly 16 break down under ambient conditions; and (4) Collection biases: phases that are difficult to 17 recognize because they lack crystal faces or are microscopic, or minerals that arise in lithological 18 contexts that are difficult to access. Minerals that conform to criterion (1), (2), or (3) are 19 inherently rare, whereas those matching criterion (4) may be much more common than 20 represented by reported occurences. 21 Rare minerals, though playing minimal roles in Earth’s bulk properties and dynamics, are 22 nevertheless of significance for varied reasons. Uncommon minerals are key to understanding 23 the diversity and disparity of Earth’s mineralogical environments, for example in the prediction 24 of as yet undescribed minerals. -
INTERNSHIP REPORT Single Crystal Growth of Constantan by Vertical
INTERNSHIP REPORT Single Crystal Growth of Constantan by Vertical Bridgman Method Supervisor: Prof. Henrik Rønnow Laboratory for Quantum Magnetism (LQM) Rahil H. Bharani 08D11004 Third year Undergraduate Metallurgical Engineering and Materials Science IIT Bombay May – July 2011 ACKNOWLEDGEMENT I thank École Polytechnique Fédérale de Lausanne (EPFL) and Prof. Henrik Rønnow, my guide, for having me as an intern here. I have always been guided with every bit of help that I could possibly require. I express my gratitude to Prof. Daniele Mari, Iva Tkalec and Ann-Kathrin Maier for helping me out with my experimental runs and providing valuable insights on several aspects of crystal growth related to the project. I thank Julian Piatek for his help in clearing any doubts that I have had regarding quantum magnetism pertaining to understanding and testing the sample. I am indebted to Neda Nikseresht and Saba Zabihzadeh for teaching me to use the SQUID magnetometer, to Nikolay Tsyrulin for the Laue Camera and Shuang Wang at PSI for the XRF in helping me analyse my samples. I thank Prof. Enrico Giannini at the University of Geneva for helping me with further trials that were conducted there. Most importantly, I thank Caroline Pletscher for helping me with every little thing that I needed and Caroline Cherpillod, Ursina Roder and Prof Pramod Rastogi for co-ordinating the entire internship program. CONTENTS INTRODUCTION REQUIREMENTS OF THE SAMPLE SOME METHODS TO GROW SINGLE CRYSTALS • CZOCHRALSKI • BRIDGMAN • FLOATING ZONE TESTING THE SAMPLES • POLISH AND ETCH • X-RAY DIFFRACTION • LAUE METHOD • SQUID • X-RAY FLUORESCENCE THE SETUP TRIAL 1 TRIAL 2 TRIAL 3 TRIAL 4 Setup, observations, results and conclusions. -
Ternary Solubility Diagrams at 270&Nbsp
Fluid Phase Equilibria 437 (2017) 1e13 Contents lists available at ScienceDirect Fluid Phase Equilibria journal homepage: www.elsevier.com/locate/fluid 2þ 2þ Partitioning of Co and Mn into meridianiite (MgSO4$11H 2O): Ternary solubility diagrams at 270 K; cation site distribution determined by single-crystal time-of-flight neutron diffraction and density functional theory * A.D. Fortes a, b, c, , I.G. Wood b, K.A. Hudson-Edwards c, M.J. Gutmann a a ISIS Facility, STFC Rutherford Appleton Laboratory, Harwell Science and Innovation Campus, Chilton, Didcot, Oxfordshire, OX11 0QX, UK b Department of Earth Sciences, University College London, Gower Street, London WC1E 6BT, UK c Department of Earth and Planetary Sciences, Birkbeck, University of London, Malet Street, London WC1E 7HX, UK article info abstract 2þ Article history: We have grown single crystals of M SO4 hydrates at 270 K from aqueous solutions in the ternary Received 14 October 2016 systems CoSO4eMgSO4eH2O and MnSO4eMgSO4eH2O. These systems exhibit broad stability fields for a Received in revised form triclinic undecahydrate on the Mg-rich side (i.e., Co- or Mn-bearing meridianiite solid solutions) and 2 January 2017 stability fields for monoclinic heptahydrates on the Mg-poor side (i.e., Mg-bearing solid solutions of Accepted 8 January 2017 bieberite or mallardite). The solubility curves and distribution coefficients, describing the partitioning of Available online 9 January 2017 þ M2 ions between liquid and solid phases, have been determined by thermo-gravimetric and spectro- þ scopic techniques. A subset of M2 SO $11H O specimens were selected for single-crystal time-of-flight Keywords: 4 2 Meridianiite neutron diffraction analysis in order to evaluate preferential occupancy of symmetry-inequivalent co- fi Epsomite ordination polyhedra in the structure. -
Phase Transition Pathways of the Hydrates of Magnesium Sulfate in the Temperature Range 50°Cto5°C: Implication for Sulfates on Mars Alian Wang,1 John J
JOURNAL OF GEOPHYSICAL RESEARCH, VOL. 114, E04010, doi:10.1029/2008JE003266, 2009 Click Here for Full Article Phase transition pathways of the hydrates of magnesium sulfate in the temperature range 50°Cto5°C: Implication for sulfates on Mars Alian Wang,1 John J. Freeman,1 and Bradley L. Jolliff1 Received 19 September 2008; revised 5 December 2008; accepted 5 January 2009; published 25 April 2009. [1] Dehydration/rehydration experiments were conducted on pure Mg-sulfates and mixtures of epsomite with Ca-sulfates, Fe-sulfates, Fe-oxide, and Fe-hydroxide. The goal was to investigate the stabilities and phase transition pathways of Mg-sulfate hydrates, under temperature and relative humidity conditions relevant to Mars, as a function of starting structure and coexisting species. Two pathways were found to form Mg-sulfate monohydrates between 5°C and 50°C through dehydration of epsomite or hexahydrite. Two polymorphs of Mg-sulfate monohydrates were characterized in this study. It is important to distinguish among these phases on Mars because they have different formation conditions that have the potential to provide additional information on surface and subsurface geologic processes. We found that Mg-sulfates with moderate hydration states (especially starkeyite and amorphous Mg-sulfates) can be very stable under current Martian surface conditions. On the basis of NIR spectral features, these phases are good candidates for polyhydrated sulfates identified on Mars by OMEGA and CRISM; thus, they may contribute to the high hydrogen concentrations found by the neutron spectrometer on the orbiting Odyssey spacecraft. Our experiments indicate that the maximum number of water molecules per SO4 held by the amorphous Mg-sulfate structure is three. -
Crystal Shape Engineering
Crystal Shape Engineering Michael A. Lovette, Andrea Robben Browning, Derek W. Gri±n, Jacob P. Sizemore, Ryan C. Snyder and Michael F. Doherty¤ Department of Chemical Engineering University of California Santa Barbara, CA 93106-5080 USA June 19, 2008 Abstract In an industrial crystallization process, crystal shape strongly influences end-product quality and functionality as well as downstream processing. Additionally, nucleation events, solvent e®ects and polymorph selection play critical roles in both the design and operation of a crystallization plant and the patentability of the product and process. Therefore, investigation of these issues with respect to a priori prediction is and will continue to be an important avenue of research. In this review, we discuss the state-of-the-art in modeling crystallization processes over a range of length scales relevant to nucleation through process design. We also identify opportunities for continued research and speci¯c areas where signi¯cant advancements are needed. ¤To whom correspondence should be addressed. Phone: (805) 893{5309 e{mail: [email protected] 1 Introduction Crystallization from solution is a process used in the chemical industries for the preparation of many types of solids (e.g., pharmaceutical products, chemical intermediates, specialty chemicals, catalysts). Several key properties of the resultant materials originate from this process, including chemical purity and composition, internal structure (polymorphic state), size and shape distribu- tions and defect density (crystallinity). Size and shape distributions impact various solid properties including end-use e±cacy (e.g., bioavailability for pharmaceuticals, reactivity for catalytics1), flowa- bility, wettability and adhesion. In turn, these properties impact down-stream processing e±ciency (e.g., ¯ltering/drying times and the possible need for milling), storage and handling. -
Chapter5 Crystallization and Characterization of a New
Propositions accompanying the thesis of F. Elif Genceli Scaling-Up Eutectic Freeze Crystallization 1. In heat flow calculations of crystallization on a cold surface [Pronk, P. et al.; Chemical Engineering Science, 2006, 61, p.4354-4362; Mershmann, A.; Crystallization Technology Handbook, 2001], it is frequently assumed that all heat of crystallization is transferred to the cold side which is not necessarily true (Chapter 7). 2. The Raman spectra presented in the work of Freeman et al. [Freeman J.J.; Wang, A.; Jolliff B.L.; 38th Lunar and Planetary Science Conference, 2007, No. 1338, p.1197] are not those of MgSO4.11H2O (Meridianiite) as they claim, but are spectra of crystal samples which have already been dehydrated into a magnesium sulfate with a lower hydrate content (Chapter 5-6). 3. It is surprising that no care was ever given since 1837 [Fritzsche, C.J.; Poggendorff’s Annalen, 1837, 42, 577-580] to determine the exact phase diagram of MgSO4 aqueous solution nor to determining the crystal structure of the magnesium sulfate phase formed at lower temperatures, despite the fact that the solution has been used in many crystallization applications as the model solution. [e.g.: Hogenboom, D.L.; Kargel, J.S.; Ganasan, J.P.; Lee, L.; Icarus. 1995, 115, 258-277] (Chapter 5). 4. It is easier to make nano-crystals from supercritical CO2 [Jung, J.; Perrut, M.; J. Supercrit. Fluids, 2001, 20, 3, 179-219] than by arrested precipitation from solution. 5. Putting CO2 underground is not a sustainable solution to our energy problem. 6. It is surprising, even paradoxical, that results in highly prestigious journals are not necessarily more reliable than those in second-tier journals. -
Alunogen Al2(SO4)3 • 17H2O C 2001-2005 Mineral Data Publishing, Version 1
Alunogen Al2(SO4)3 • 17H2O c 2001-2005 Mineral Data Publishing, version 1 Crystal Data: Triclinic. Point Group: 1. Crystals rare, as thin plates or prismatic [001] or {010} with a six-sided outline about [010], very complex, with about 60 forms noted. Commonly fibrous, to 5 mm, forming delicate masses, crusts, and efflorescences. Twinning: On {010}. Physical Properties: Cleavage: {010}, perfect; probable on {100} and {313}. Hardness = 1.5–2 D(meas.) = 1.72–1.77 D(calc.) = 1.79 Soluble in H2O; taste alumlike, acid and sharp. Optical Properties: Transparent. Color: Colorless in crystals, aggregates white, or pale yellow or red from impurities; colorless in transmitted light. Luster: Vitreous to silky. Optical Class: Biaxial (+). Orientation: X ' b; Z ∧ c =42◦. α = 1.459–1.475 β = 1.461–1.478 γ = 1.470–1.485 2V(meas.) = 31◦ Cell Data: Space Group: P 1. a = 7.420(6) b = 26.97(2) c = 6.062(5) α =89◦57(5)0 β =97◦34(5)0 γ =91◦53(5)0 Z=2 X-ray Powder Pattern: Nov´aBaˇna, Slovakia. 4.489 (100), 4.390 (81), 3.969 (81), 4.329 (76), 13.46 (54), 3.897 (52), 3.675 (45) Chemistry: (1) (2) SO3 37.74 37.04 Al2O3 16.59 15.73 H2O 44.64 47.23 insol. 0.94 Total 99.91 100.00 • (1) Pintado Canyon, Guadalupe Co., New Mexico, USA. (2) Al2(SO4)3 17H2O. Occurrence: Forms by reaction of sulfates from decomposing sulfides with aluminous minerals in shales and slates; in gossan or altered wall rock of pyritic deposits in arid regions; in coal seams; in relatively low-temperature fumaroles.