Chapter 6 Target Selection
Total Page:16
File Type:pdf, Size:1020Kb
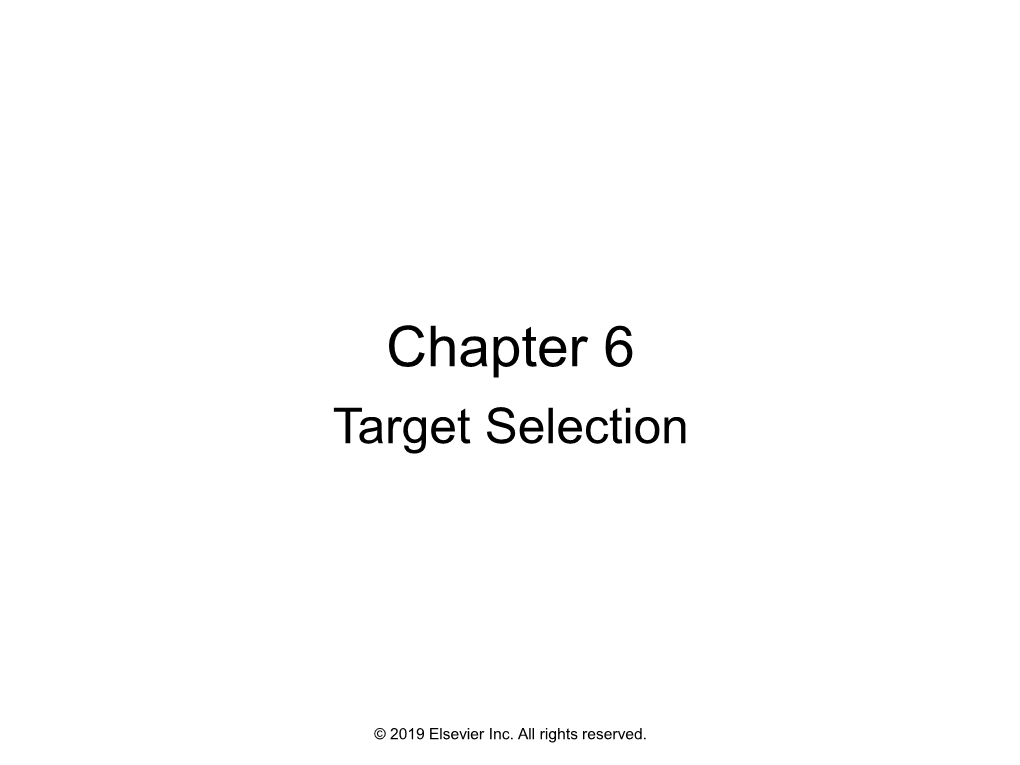
Load more
Recommended publications
-
Understanding Axon Guidance: Are We Nearly There Yet?
Zurich Open Repository and Archive University of Zurich Main Library Strickhofstrasse 39 CH-8057 Zurich www.zora.uzh.ch Year: 2018 Understanding axon guidance: are we nearly there yet? Stoeckli, Esther T Abstract: During nervous system development, neurons extend axons to reach their targets and form functional circuits. The faulty assembly or disintegration of such circuits results in disorders of the nervous system. Thus, understanding the molecular mechanisms that guide axons and lead to neural circuit formation is of interest not only to developmental neuroscientists but also for a better comprehension of neural disorders. Recent studies have demonstrated how crosstalk between different families of guidance receptors can regulate axonal navigation at choice points, and how changes in growth cone behaviour at intermediate targets require changes in the surface expression of receptors. These changes can be achieved by a variety of mechanisms, including transcription, translation, protein-protein interactions, and the specific trafficking of proteins and mRNAs. Here, I review these axon guidance mechanisms, highlighting the most recent advances in the field that challenge the textbook model of axon guidance. DOI: https://doi.org/10.1242/dev.151415 Posted at the Zurich Open Repository and Archive, University of Zurich ZORA URL: https://doi.org/10.5167/uzh-166034 Journal Article Published Version Originally published at: Stoeckli, Esther T (2018). Understanding axon guidance: are we nearly there yet? Development, 145(10):dev151415. DOI: https://doi.org/10.1242/dev.151415 © 2018. Published by The Company of Biologists Ltd | Development (2018) 145, dev151415. doi:10.1242/dev.151415 REVIEW Understanding axon guidance: are we nearly there yet? Esther T. -
Lysosomal Function and Axon Guidance: Is There a Meaningful Liaison?
biomolecules Review Lysosomal Function and Axon Guidance: Is There a Meaningful Liaison? Rosa Manzoli 1,2,†, Lorenzo Badenetti 1,3,4,†, Michela Rubin 1 and Enrico Moro 1,* 1 Department of Molecular Medicine, University of Padova, 35121 Padova, Italy; [email protected] (R.M.); [email protected] (L.B.); [email protected] (M.R.) 2 Department of Biology, University of Padova, 35121 Padova, Italy 3 Department of Women’s and Children’s Health, University of Padova, 35121 Padova, Italy 4 Pediatric Research Institute “Città della Speranza”, 35127 Padova, Italy * Correspondence: [email protected]; Tel.: +39-04-98276341 † These authors contributed equally to this paper. Abstract: Axonal trajectories and neural circuit activities strongly rely on a complex system of molec- ular cues that finely orchestrate the patterning of neural commissures. Several of these axon guidance molecules undergo continuous recycling during brain development, according to incompletely un- derstood intracellular mechanisms, that in part rely on endocytic and autophagic cascades. Based on their pivotal role in both pathways, lysosomes are emerging as a key hub in the sophisticated regulation of axonal guidance cue delivery, localization, and function. In this review, we will attempt to collect some of the most relevant research on the tight connection between lysosomal function and axon guidance regulation, providing some proof of concepts that may be helpful to understanding the relation between lysosomal storage disorders and neurodegenerative diseases. Citation: Manzoli, R.; Badenetti, L.; Keywords: axon guidance; lysosomal storage disorders; neuronal circuit Rubin, M.; Moro, E. Lysosomal Function and Axon Guidance: Is There a Meaningful Liaison? Biomolecules 2021, 11, 191. -
Retinal Ganglion Cell Axon Sorting at the Optic Chiasm Requires Dystroglycan
bioRxiv preprint doi: https://doi.org/10.1101/286005; this version posted March 21, 2018. The copyright holder for this preprint (which was not certified by peer review) is the author/funder. All rights reserved. No reuse allowed without permission. Retinal Ganglion Cell Axon Sorting at the Optic Chiasm Requires Dystroglycan Reena Clements1 and Kevin M. Wright1,2, # 1) Neuroscience Graduate Program, 2) Vollum Institute, Oregon Health & Science University, Portland, OR 97239, USA #) Correspondence to Kevin M. Wright ([email protected]). Vollum Institute, 3181 SW Sam Jackson Park Rd L474 Portland, OR 97239. (503)-494-6955 Running title: Dystroglycan regulates RGC sorting Key Words: dystroglycan, axon, optic chiasm, retina, basement membrane, extracellular matrix bioRxiv preprint doi: https://doi.org/10.1101/286005; this version posted March 21, 2018. The copyright holder for this preprint (which was not certified by peer review) is the author/funder. All rights reserved. No reuse allowed without permission. 1 Summary Statement 2 Abnormal retinal ganglion cell axon sorting in the optic chiasm in the absence of 3 functional dystroglycan results in profound defects in retinorecipient innervation. 4 5 Abstract 6 In the developing visual system, retinal ganglion cell (RGC) axons project 7 from the retina to several distal retinorecipient regions in the brain. Several 8 molecules have been implicated in guiding RGC axons in vivo, but the role of 9 extracellular matrix molecules in this process remains poorly understood. 10 Dystroglycan is a laminin-binding transmembrane protein important for formation 11 and maintenance of the extracellular matrix and basement membranes and has 12 previously been implicated in axon guidance in the developing spinal cord. -
Master Layout Sheet
2893 REVIEW ARTICLE CANADIAN ASSOCIATION OF NEUROSCIENCES REVIEW: Postnatal Development of the Mammalian Neocortex: Role of Activity Revisited Zhong-wei Zhang ABSTRACT: The mammalian neocortex is the largest structure in the brain, and plays a key role in brain function. A critical period for the development of the neocortex is the early postnatal life, when the majority of synapses are formed and when much of synaptic remodeling takes place. Early studies suggest that initial synaptic connections lack precision, and this rudimentary wiring pattern is refined by experience-related activity through selective elimination and consolidation. This view has been challenged by recent studies revealing the presence of a relatively precise pattern of connections before the onset of sensory experience. The recent data support a model in which specificity of neuronal connections is largely determined by genetic factors. Spontaneous activity is required for the formation of neural circuits, but whether it plays an instructive role is still controversial. Neurotransmitters including acetylcholine, serotonin, and γ-Aminobutyric acid (GABA) may have key roles in the regulation of spontaneous activity, and in the maturation of synapses in the developing brain. RÉSUMÉ: Développement postnatal du néocortex chez les mammifères: révision du rôle de l’activité. Le néocortex des mammifères est la plus grosse structure du cerveau et il joue un rôle stratégique dans la fonction cérébrale. La période postnatale est critique pour son développement. La majorité des synapses se forment à ce moment-là ainsi qu’une grande partie du remodelage synaptique. Plusieurs études ont suggéré que les connections synaptiques initiales manquent de précision et que ce « câblage » rudimentaire du cerveau est raffiné par l’activité reliée à l’expérience, par élimination et consolidation sélective. -
Molecular Regulation of Visual System Development: More Than Meets the Eye
Downloaded from genesdev.cshlp.org on September 30, 2021 - Published by Cold Spring Harbor Laboratory Press REVIEW Molecular regulation of visual system development: more than meets the eye Takayuki Harada,1,2 Chikako Harada,1,2 and Luis F. Parada1,3 1Department of Developmental Biology and Kent Waldrep Foundation Center for Basic Neuroscience Research on Nerve Growth and Regeneration, University of Texas Southwestern Medical Center, Dallas, Texas 75235, USA; 2Department of Molecular Neurobiology, Tokyo Metropolitan Institute for Neuroscience, Fuchu, Tokyo 183-8526, Japan Vertebrate eye development has been an excellent model toderm, intercalating mesoderm, surface ectoderm, and system to investigate basic concepts of developmental neural crest (Fig. 1). The neuroectoderm differentiates biology ranging from mechanisms of tissue induction to into the retina, iris, and optic nerve; the surface ecto- the complex patterning and bidimensional orientation of derm gives rise to lens and corneal epithelium; the me- the highly specialized retina. Recent advances have shed soderm differentiates into the extraocular muscles and light on the interplay between numerous transcriptional the fibrous and vascular coats of the eye; and neural crest networks and growth factors that are involved in the cells become the corneal stroma sclera and corneal en- specific stages of retinogenesis, optic nerve formation, dothelium. The vertebrate eye originates from bilateral and topographic mapping. In this review, we summarize telencephalic optic grooves. In humans, optic vesicles this recent progress on the molecular mechanisms un- emerge at the end of the fourth week of development and derlying the development of the eye, visual system, and soon thereafter contact the surface ectoderm to induce embryonic tumors that arise in the optic system. -
Axon Guidance Molecules in Vascular Patterning
Downloaded from http://cshperspectives.cshlp.org/ on September 26, 2021 - Published by Cold Spring Harbor Laboratory Press Axon Guidance Molecules in Vascular Patterning Ralf H. Adams1 and Anne Eichmann2 1Max-Planck-Institute for Molecular Biomedicine, Department of Tissue Morphogenesis, and University of Mu¨nster, Faculty of Medicine, Mu¨nster, Germany 2Inserm U833, College de France, 11 Place Marcelin Berthelot, 75005 Paris, France Correspondence: [email protected] Endothelial cells (ECs) form extensive, highly branched and hierarchically organized tubular networks in vertebrates to ensure the proper distribution of molecular and cellular cargo in the vertebrate body. The growth of this vascular system during development, tissue repair or in disease conditions involves the sprouting, migration and proliferation of endothelial cells in a process termed angiogenesis. Surprisingly, specialized ECs, so-called tip cells, which lead and guide endothelial sprouts, share many feature with another guidance struc- ture, the axonal growth cone. Tip cells are motile, invasive and extend numerous filopodial protrusions sensing growth factors, extracellular matrix and other attractive or repulsive cues in their tissue environment. Axonal growth cones and endothelial tip cells also respond to signals belonging to the same molecular families, such as Slits and Roundabouts, Netrins and UNC5 receptors, Semaphorins, Plexins and Neuropilins, and Eph receptors and ephrin ligands. Here we summarize fundamental principles of angiogenic growth, the selec- tion and function of tip cells and the underlying regulation by guidance cues, the Notch pathway and vascular endothelial growth factor signaling. PATTERNING OF THE VASCULAR SYSTEM The circulating blood travels from the heart he vasculature, like the nervous system, through the aorta, the largest axial vessel, into Tforms a highly branched, tree-like network a hierarchical system of arteries and smaller that reaches into every organ of vertebrate arterioles into distal capillary beds (Fig. -
Studying the Role of Axon Fasciculation During
www.nature.com/scientificreports OPEN Studying the role of axon fasciculation during development in a computational model of the Received: 3 July 2017 Accepted: 3 October 2017 Xenopus tadpole spinal cord Published: xx xx xxxx Oliver Davis1, Robert Merrison-Hort 2, Stephen R. Sofe3 & Roman Borisyuk2,4 During nervous system development growing axons can interact with each other, for example by adhering together in order to produce bundles (fasciculation). How does such axon-axon interaction afect the resulting axonal trajectories, and what are the possible benefts of this process in terms of network function? In this paper we study these questions by adapting an existing computational model of the development of neurons in the Xenopus tadpole spinal cord to include interactions between axons. We demonstrate that even relatively weak attraction causes bundles to appear, while if axons weakly repulse each other their trajectories diverge such that they fll the available space. We show how fasciculation can help to ensure axons grow in the correct location for proper network formation when normal growth barriers contain gaps, and use a functional spiking model to show that fasciculation allows the network to generate reliable swimming behaviour even when overall synapse counts are artifcially lowered. Although we study fasciculation in one particular organism, our approach to modelling axon growth is general and can be widely applied to study other nervous systems. In this paper we describe a computational model of the anatomy and functionality of neuronal networks in the spinal cord of the hatchling Xenopus laevis tadpole. Tis study is based on extensive previous research on axon growth1–3 and neuronal activity4 in this relatively simple and well-understood system. -
Axon Guidance I
Axon guidance I Paul Garrity March 15, 2004 7.68/9.013 Neuronal Wiring: Functional Framework of the Nervous System Stretch reflex circuit Early theories of axonogenesis • Schwann: many neurons link to form a chain • Hensen: axon forms around preexisitng threads between cells • Wilhelm His (1886) and Santiago Ramon y Cajal (1890): Proposed that axon is an outgrowth from a neuron Axon outgrowth • Ross Harrison (1907):Invented tissue culture to demonstrate axon extension – Isolated piece of neural tube from tadpole – Placed neuroblasts in drop of frog lymph on coverslip inverted over depression slide – Watched axons emerge from differentiating neurons in his “hanging drop” prep at 56 microns/hour Adapted from Harrison (1908) The growth cone • At leading edge of the axon (and dendrite) is a motile structure where much of the control of axon navigation takes place: the growth cone Growth cones are highly dynamic • Growth cones crawl over a variety of surfaces to reach their targets and have a wide variety of shapes in vivo Ramon y Cajal 1890 Movies of axon guidance in vivo • Xenopus spinal cord – http://gomez.anatomy.wisc.edu/Lab%20Page% 20folder/Lab%20Page/SpinalCord.html » 5 min/frame; 5 hours; • Xenopus visual system – http://www.anat.cam.ac.uk/pages/staff/academi c/holt/large.mov » 3 min/frame; 6 hours Neurons show cell-type specific axon projection patterns An axon’s complex journey can be broken into discrete segments • Axons navigate using a series of intermediate targets – Example: Ti1 neuron in grasshopper limb bud Intermediate targets -
Retinal Ganglion Cell Axon Guidance in the Mouse Optic Chiasm: Expression and Function of Robos and Slits
The Journal of Neuroscience, July 1, 2000, 20(13):4975–4982 Retinal Ganglion Cell Axon Guidance in the Mouse Optic Chiasm: Expression and Function of Robos and Slits Lynda Erskine,1 Scott E. Williams,1 Katja Brose,2 Thomas Kidd,3 Rivka A. Rachel,1 Corey S. Goodman,3 Marc Tessier-Lavigne,2 and Carol A. Mason1 1Departments of Pathology and Anatomy and Cell Biology, Center for Neurobiology and Behavior, Columbia University, College of Physicians and Surgeons, New York, New York 10032, 2Howard Hughes Medical Institute, Departments of Anatomy, and Biochemistry and Biophysics, University of California, San Francisco, California, 94143-0452, and 3Howard Hughes Medical Institute, Department of Molecular and Cell Biology, University of California, Berkeley, California 94720 The ventral midline of the nervous system is an important brain. The receptors and ligands are expressed at the appro- choice point at which growing axons decide whether to cross priate time and place, in both the retina and the ventral dien- and project contralaterally or remain on the same side of the cephalon, to be able to influence RGC axon guidance. In vitro, brain. In Drosophila, the decision to cross or avoid the CNS slit2 is inhibitory to RGC axons, with outgrowth of both ipsilat- midline is controlled, at least in part, by the Roundabout (Robo) erally and contralaterally projecting axons being strongly af- receptor on the axons and its ligand, Slit, an inhibitory extra- fected. Overall, these results indicate that Robos and Slits cellular matrix molecule secreted by the midline glia. Vertebrate alone do not directly control RGC axon divergence at the optic homologs of these molecules have been cloned and have also chiasm and may additionally function as a general inhibitory been implicated in regulating axon guidance. -
The Molecular Biology of Axon Guidance -- Tessier-Lavigne and Goodman 274 (5290): 1123 -- Science
The Molecular Biology of Axon Guidance -- Tessier-Lavigne and Goodman 274 (5290): 1123 -- Science Science 15 November 1996: Vol. 274. no. 5290, pp. 1123 - 1133 Prev | Table of Contents | Next DOI: 10.1126/science.274.5290.1123 Articles The Molecular Biology of Axon Guidance Marc Tessier-Lavigne and Corey S. Goodman Neuronal growth cones navigate over long distances along specific pathways to find their correct targets. The mechanisms and molecules that direct this pathfinding are the topics of this review. Growth cones appear to be guided by at least four different mechanisms: contact attraction, chemoattraction, contact repulsion, and chemorepulsion. Evidence is accumulating that these mechanisms act simultaneously and in a coordinated manner to direct pathfinding and that they are mediated by mechanistically and evolutionarily conserved ligand-receptor systems. M. Tessier-Lavigne is in the Department of Anatomy, Howard Hughes Medical Institute, University of California, San Francisco, CA 94143, USA. C. S. Goodman is in the Department of Molecular and Cell Biology, Howard Hughes Medical Institute, University of California, Berkeley, CA 94720, USA. The remarkable feats of information-processing performed by the brain are determined to a large extent by the intricate network of connections between nerve cells (or neurons). The magnitude of the task involved in wiring the nervous system is staggering. In adult humans, each of over a trillion neurons makes connections with, on average, over a thousand target cells, in an intricate circuit whose precise pattern is essential for the proper functioning of the nervous system. How can this pattern be generated during embryogenesis with the necessary precision and reliability? Neuronal connections form during embryonic development when each differentiating neuron sends out an axon, tipped at its leading edge by the growth cone, which migrates through the embryonic environment to its synaptic targets, laying down the extending axon in its wake (Fig. -
The Genetics of Axon Guidance and Axon Regeneration in Caenorhabditis Elegans
| WORMBOOK NEUROBIOLOGY AND BEHAVIOR The Genetics of Axon Guidance and Axon Regeneration in Caenorhabditis elegans Andrew D. Chisholm,*,1 Harald Hutter,†,1 Yishi Jin,*,‡,§,1 and William G. Wadsworth**,1 *Section of Neurobiology, Division of Biological Sciences, and ‡Department of Cellular and Molecular Medicine, School of Medicine, University of California, San Diego, La Jolla, California 92093, †Department of Biological Sciences, Simon Fraser University, Burnaby, British Columbia, V5A 1S6, Canada, §Department of Pathology and Laboratory Medicine, Howard Hughes Medical Institute, Chevy Chase, Maryland, and **Department of Pathology, Rutgers Robert Wood Johnson Medical School, Piscataway, New Jersey 08854 ORCID IDs: 0000-0001-5091-0537 (A.D.C.); 0000-0002-9371-9860 (Y.J.); 0000-0003-3824-2948 (W.G.W.) ABSTRACT The correct wiring of neuronal circuits depends on outgrowth and guidance of neuronal processes during development. In the past two decades, great progress has been made in understanding the molecular basis of axon outgrowth and guidance. Genetic analysis in Caenorhabditis elegans has played a key role in elucidating conserved pathways regulating axon guidance, including Netrin signaling, the slit Slit/Robo pathway, Wnt signaling, and others. Axon guidance factors were first identified by screens for mutations affecting animal behavior, and by direct visual screens for axon guidance defects. Genetic analysis of these pathways has revealed the complex and combinatorial nature of guidance cues, and has delineated how cues guide growth cones via receptor activity and cytoskeletal rearrangement. Several axon guidance pathways also affect directed migrations of non-neuronal cells in C. elegans, with implications for normal and pathological cell migrations in situations such as tumor metastasis. -
Slit Inhibition of Retinal Axon Growth and Its Role in Retinal Axon Pathfinding and Innervation Patterns in the Diencephalon
The Journal of Neuroscience, July 1, 2000, 20(13):4983–4991 Slit Inhibition of Retinal Axon Growth and Its Role in Retinal Axon Pathfinding and Innervation Patterns in the Diencephalon Thomas Ringstedt,1 Janet E. Braisted,1 Katja Brose,2 Thomas Kidd,3 Corey Goodman,3 Marc Tessier-Lavigne,2 and Dennis D. M. O’Leary1 1Molecular Neurobiology Laboratory, The Salk Institute, La Jolla, California 92037, 2Departments of Anatomy, and Biochemistry and Biophysics, University of California, San Francisco, California 94143-0452, and 3Department of Molecular and Cell Biology, University of California, Berkeley, California 94720 We have analyzed the role of the Slit family of repellent axon retinal axons are more tightly fasciculated. This action of Slit2 guidance molecules in the patterning of the axonal projections as a growth inhibitor of retinal axons and the expression pat- of retinal ganglion cells (RGCs) within the embryonic rat dien- terns of slit1 and slit2 correlate with the fasciculation and cephalon and whether the slits can account for a repellent innervation patterns of RGC axons within the diencephalon and activity for retinal axons released by hypothalamus and epith- implicate the Slits as components of the axon repellent activity alamus. At the time RGC axons extend over the diencephalon, associated with the hypothalamus and epithalamus. Our find- slit1 and slit2 are expressed in hypothalamus and epithalamus ings suggest that in vivo the Slits control RGC axon pathfinding but not in the lateral part of dorsal thalamus, a retinal target. and targeting within the diencephalon by regulating their fas- slit3 expression is low or undetectable. The Slit receptors ciculation, preventing them or their branches from invading robo2, and to a limited extent robo1, are expressed in the RGC nontarget tissues, and steering them toward their most distal layer, as are slit1 and slit2.