CHARACTERIZATION of Arthrobacter Globiformis ASPARTATE
Total Page:16
File Type:pdf, Size:1020Kb
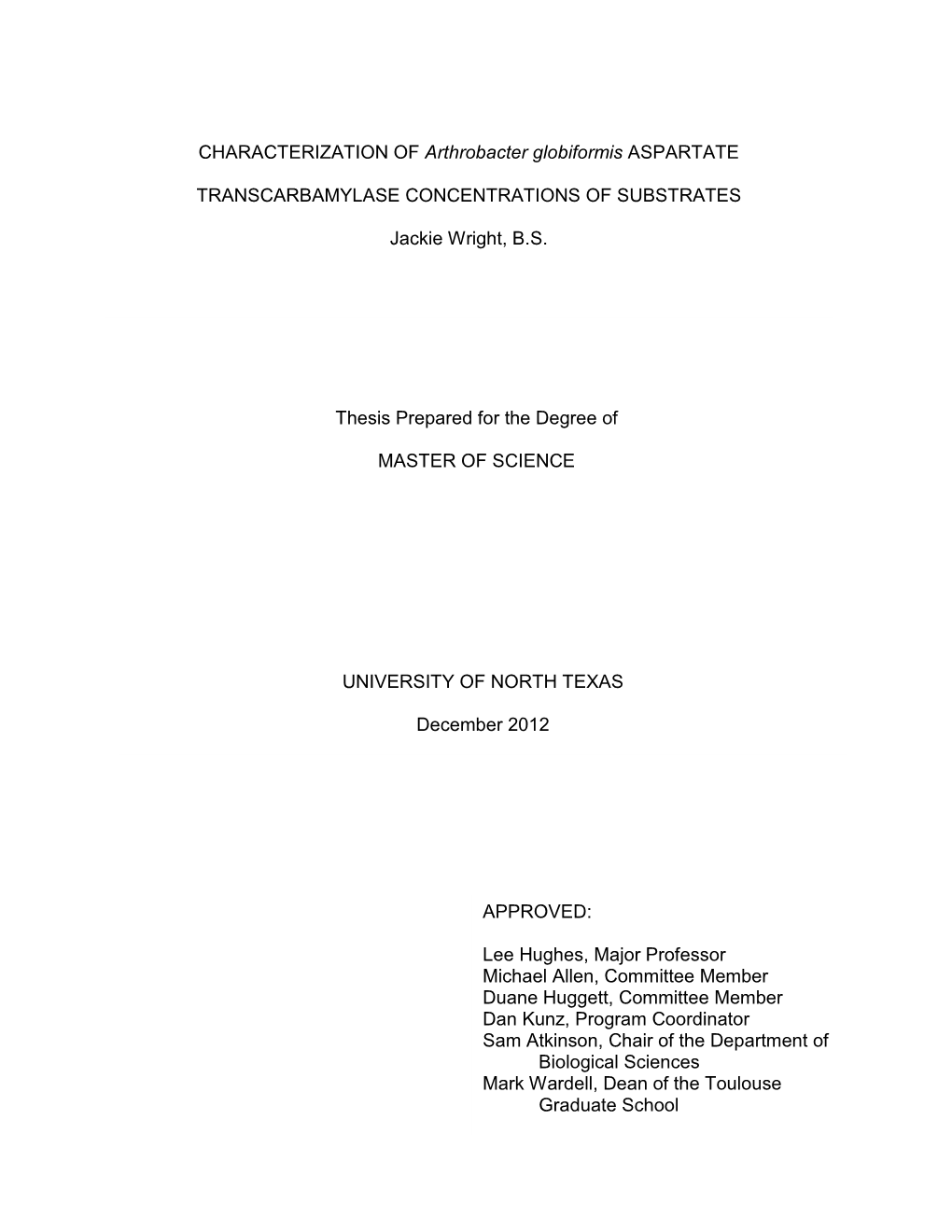
Load more
Recommended publications
-
Light-Induced Psba Translation in Plants Is Triggered by Photosystem II Damage Via an Assembly-Linked Autoregulatory Circuit
Light-induced psbA translation in plants is triggered by photosystem II damage via an assembly-linked autoregulatory circuit Prakitchai Chotewutmontria and Alice Barkana,1 aInstitute of Molecular Biology, University of Oregon, Eugene, OR 97403 Edited by Krishna K. Niyogi, University of California, Berkeley, CA, and approved July 22, 2020 (received for review April 26, 2020) The D1 reaction center protein of photosystem II (PSII) is subject to mRNA to provide D1 for PSII repair remain obscure (13, 14). light-induced damage. Degradation of damaged D1 and its re- The consensus view in recent years has been that psbA transla- placement by nascent D1 are at the heart of a PSII repair cycle, tion for PSII repair is regulated at the elongation step (7, 15–17), without which photosynthesis is inhibited. In mature plant chloro- a view that arises primarily from experiments with the green alga plasts, light stimulates the recruitment of ribosomes specifically to Chlamydomonas reinhardtii (Chlamydomonas) (18). However, we psbA mRNA to provide nascent D1 for PSII repair and also triggers showed recently that regulated translation initiation makes a a global increase in translation elongation rate. The light-induced large contribution in plants (19). These experiments used ribo- signals that initiate these responses are unclear. We present action some profiling (ribo-seq) to monitor ribosome occupancy on spectrum and genetic data indicating that the light-induced re- cruitment of ribosomes to psbA mRNA is triggered by D1 photo- chloroplast open reading frames (ORFs) in maize and Arabi- damage, whereas the global stimulation of translation elongation dopsis upon shifting seedlings harboring mature chloroplasts is triggered by photosynthetic electron transport. -
Etude Des Sources De Carbone Et D'énergie Pour La Synthèse Des Lipides De Stockage Chez La Microalgue Verte Modèle Chlamydo
Aix Marseille Université L'Ecole Doctorale 62 « Sciences de la Vie et de la Santé » Etude des sources de carbone et d’énergie pour la synthèse des lipides de stockage chez la microalgue verte modèle Chlamydomonas reinhardtii Yuanxue LIANG Soutenue publiquement le 17 janvier 2019 pour obtenir le grade de « Docteur en biologie » Jury Professor Claire REMACLE, Université de Liège (Rapporteuse) Dr. David DAUVILLEE, CNRS Lille (Rapporteur) Professor Stefano CAFFARRI, Aix Marseille Université (Examinateur) Dr. Gilles PELTIER, CEA Cadarache (Invité) Dr. Yonghua LI-BEISSON, CEA Cadarache (Directeur de thèse) 1 ACKNOWLEDGEMENTS First and foremost, I would like to express my sincere gratitude to my advisor Dr. Yonghua Li-Beisson for the continuous support during my PhD study and also gave me much help in daily life, for her patience, motivation and immense knowledge. I could not have imagined having a better mentor. I’m also thankful for the opportunity she gave me to conduct my PhD research in an excellent laboratory and in the HelioBiotec platform. I would also like to thank another three important scientists: Dr. Gilles Peltier (co- supervisor), Dr. Fred Beisson and Dr. Pierre Richaud who helped me in various aspects of the project. I’m not only thankful for their insightful comments, suggestion, help and encouragement, but also for the hard question which incented me to widen my research from various perspectives. I would also like to thank collaboration from Fantao, Emmannuelle, Yariv, Saleh, and Alisdair. Fantao taught me how to cultivate and work with Chlamydomonas. Emmannuelle performed bioinformatic analyses. Yariv, Saleh and Alisdair from Potsdam for amino acid analysis. -
The Regulation of Carbamoyl Phosphate Synthetase-Aspartate Transcarbamoylase-Dihydroorotase (Cad) by Phosphorylation and Protein-Protein Interactions
THE REGULATION OF CARBAMOYL PHOSPHATE SYNTHETASE-ASPARTATE TRANSCARBAMOYLASE-DIHYDROOROTASE (CAD) BY PHOSPHORYLATION AND PROTEIN-PROTEIN INTERACTIONS Eric M. Wauson A dissertation submitted to the faculty of the University of North Carolina at Chapel Hill in partial fulfillment of the requirements for the degree of Doctor of Philosophy in the Department of Pharmacology. Chapel Hill 2007 Approved by: Lee M. Graves, Ph.D. T. Kendall Harden, Ph.D. Gary L. Johnson, Ph.D. Aziz Sancar M.D., Ph.D. Beverly S. Mitchell, M.D. 2007 Eric M. Wauson ALL RIGHTS RESERVED ii ABSTRACT Eric M. Wauson: The Regulation of Carbamoyl Phosphate Synthetase-Aspartate Transcarbamoylase-Dihydroorotase (CAD) by Phosphorylation and Protein-Protein Interactions (Under the direction of Lee M. Graves, Ph.D.) Pyrimidines have many important roles in cellular physiology, as they are used in the formation of DNA, RNA, phospholipids, and pyrimidine sugars. The first rate- limiting step in the de novo pyrimidine synthesis pathway is catalyzed by the carbamoyl phosphate synthetase II (CPSase II) part of the multienzymatic complex Carbamoyl phosphate synthetase, Aspartate transcarbamoylase, Dihydroorotase (CAD). CAD gene induction is highly correlated to cell proliferation. Additionally, CAD is allosterically inhibited or activated by uridine triphosphate (UTP) or phosphoribosyl pyrophosphate (PRPP), respectively. The phosphorylation of CAD by PKA and ERK has been reported to modulate the response of CAD to allosteric modulators. While there has been much speculation on the identity of CAD phosphorylation sites, no definitive identification of in vivo CAD phosphorylation sites has been performed. Therefore, we sought to determine the specific CAD residues phosphorylated by ERK and PKA in intact cells. -
Maturation of Sucrase-Isomaltase Complex in Human Fetal Small and Large Intestine During Gestation
136 TRIADOU AND ZWEIBAUM 003 1-3998/85/190 1-0 136$02.0010 PEDIATRIC RESEARCH Vol. 19, No. 1, 1985 Copyright O 1985 International Pediatric Research Foundation, Inc Prin~edin U.S. A. Maturation of Sucrase-Isomaltase Complex in Human Fetal Small and Large Intestine during Gestation NICOLE TRIADOU AND ALAIN ZWEIBAUM UnitP de Recherches de GPnetique MPdicale [N. T.], INSERM U 12, H6pital des Enfants Malades, and Unite de Recherches sur le MPtabolisme et la Diffirmciation des Cellules en Culture [A.Z.],INSERM U 178, H6pital Broussais, Paris, France ABSTRACT. Sucrase-isomaltase complex is expressed in chromatography. The homogeneity of the preparation was as- human small intestine throughout gestation and in the large sessed by polyacrylamide gel electrophoresis and crossed immu- intestine between 12 and 30 wk. The molecular form of the noelectrophoresis against an antihuman brush-border antiserum enzyme was studied in the brush-border membrane frac- (12). Rabbits were immunized by sc injections at 15-day intervals tions by the immunoblotting method. Before 30 wk of with 0.2 mg of purified enzyme and bled 7 days after the fourth gestation, the enzyme is present only as the high molecular injection. Immunoglobulins G were prepared by ion exchange weight prosucrase-isomaltase, while from 30 wk until birth chromatography (10, 15). the two subunits are also present. The fetal enzyme, as its Brush-border separation, transjer, and identification of antigen. proform and as its two subunits, has a faster mobility in Preparations of the brush-border fractions of small and large sodium-dodecylsulfate polyacrylamide gel electrophoresis, intestine were obtained by the calcium precipitation method as than the adult enzyme (removal of sialic acid residues from previously described (12). -
(CP) Gene of Papaya Ri
Results and Discussion 4. RESULTS AND DISCUSSION 4.1 Genetic diversity analysis of coat protein (CP) gene of Papaya ringspot virus-P (PRSV-P) isolates from multiple locations of Western India Results – 4.1.1 Sequence analysis In this study, fourteen CP gene sequences of PRSV-P originating from multiple locations of Western Indian States, Gujarat and Maharashtra (Fig. 3.1), have been analyzed and compared with 46 other CP sequences from different geographic locations of America (8), Australia (1), Asia (13) and India (24) (Table 4.1; Fig. 4.1). The CP length of the present isolates varies from 855 to 861 nucleotides encoding 285 to 287 amino acids. Fig. 4.1: Amplification of PRSV-P coat protein (CP) gene from 14 isolates of Western India. From left to right lanes:1: Ladder (1Kb), 2: IN-GU-JN, 3: IN-GU-SU, 4: IN-GU-DS, 5: IN-GU-RM, 6: IN-GU-VL, 7: IN-MH-PN, 8: IN-MH-KO, 9: IN-MH-PL, 10: IN-MH-SN, 11: IN-MH-JL, 12: IN-MH-AM, 13: IN-MH-AM, 14: IN-MH-AK, 15: IN-MH-NS,16: Negative control. Red arrow indicates amplicon of Coat protein (CP) gene. Table 4.1: Sources of coat protein (CP) gene sequences of PRSV-P isolates from India and other countries used in this study. Country Name of Length GenBank Origin¥ Reference isolates* (nts) Acc No IN-GU-JN GU-Jamnagar 861 MG977140 This study IN-GU-SU GU-Surat 855 MG977142 This study IN-GU-DS GU-Desalpur 855 MG977139 This study India IN-GU-RM GU-Ratlam 858 MG977141 This study IN-GU-VL GU-Valsad 855 MG977143 This study IN-MH-PU MH-Pune 861 MH311882 This study Page | 36 Results and Discussion IN-MH-PN MH-Pune -
Itraq-Based Proteome Profiling Revealed the Role of Phytochrome A
www.nature.com/scientificreports OPEN iTRAQ‑based proteome profling revealed the role of Phytochrome A in regulating primary metabolism in tomato seedling Sherinmol Thomas1, Rakesh Kumar2,3, Kapil Sharma2, Abhilash Barpanda1, Yellamaraju Sreelakshmi2, Rameshwar Sharma2 & Sanjeeva Srivastava1* In plants, during growth and development, photoreceptors monitor fuctuations in their environment and adjust their metabolism as a strategy of surveillance. Phytochromes (Phys) play an essential role in plant growth and development, from germination to fruit development. FR‑light (FR) insensitive mutant (fri) carries a recessive mutation in Phytochrome A and is characterized by the failure to de‑etiolate in continuous FR. Here we used iTRAQ‑based quantitative proteomics along with metabolomics to unravel the role of Phytochrome A in regulating central metabolism in tomato seedlings grown under FR. Our results indicate that Phytochrome A has a predominant role in FR‑mediated establishment of the mature seedling proteome. Further, we observed temporal regulation in the expression of several of the late response proteins associated with central metabolism. The proteomics investigations identifed a decreased abundance of enzymes involved in photosynthesis and carbon fxation in the mutant. Profound accumulation of storage proteins in the mutant ascertained the possible conversion of sugars into storage material instead of being used or the retention of an earlier profle associated with the mature embryo. The enhanced accumulation of organic sugars in the seedlings indicates the absence of photomorphogenesis in the mutant. Plant development is intimately bound to the external light environment. Light drives photosynthetic carbon fxa- tion and activates a set of signal-transducing photoreceptors that regulate plant growth and development. -
CV – Professor Toshiki ENOMOTO
CV – Professor Toshiki ENOMOTO Name: Toshiki ENOMOTO, Ph.D. Date of Birth: May 14th 1958 Nationality: Japanese Present Position: Professor of Department of Food Science (Food Chemistry Laboratory), Faculty of Bioresources and Environmental Sciences, Ishikawa Prefectural University Office Address 1-308 Suematsu, Nonoichi, Ishikawa 921-8836, Japan Tel and Fax: +81-76-227-7452 E-mail: [email protected] Educational Background: 1983 Received BS. From Dept of Animal Science, Obihiro University of Agriculture and Veterinary Medicine 1985 Received MS. From Graduate School of Agricultural Science, Osaka Prefecture University 1988 Received PhD. From Graduate School of Agricultural Science, Osaka Prefecture University Research Field: Food Chemistry and Food Function Research Themes in 2019 1. Identification of oligosaccharides in honey 2. The volatile compounds of Kaga boucya (roasted stem tea) 3. Chemical composition and microflora of Asian fermented fish products 4. Anti-influenza virus activity of agricultural, forestry and fishery products 5. Chemical composition and microflora of kefir 6. Elucidation of detoxification mechanism of tetrodotoxin from puffer fish ovaries picked in rice bran Professional Carrier: 1988 Assistant Professor, Division of Life and Health Sciences, 1 Joetsu University of Education 1993 Associate Professor, Department of Food Science, Ishikawa Agricultural College 1994-1995 Visiting Scientist Supported by Ministry of Education, Culture, Sports, Science and Technology-Japan, School of Biological Sciences, University -
Supplementary Material Title Comparative Proteomic Analysis Of
Supplementary Material Title Comparative proteomic analysis of wild-type Physcomitrella patens and an OPDA-deficient Physcomitrella patens mutant with disrupted PpAOS1 and PpAOS2 genes after wounding Authors Weifeng Luoa, Setsuko Komatsub, Tatsuya Abea, Hideyuki Matsuuraa, and Kosaku Takahashia,c* Affiliations aResearch Faculty of Agriculture, Hokkaido University, Sapporo 606-8589, Japan bDepartment of Environmental and Food Sciences, Faculty of Environmental and Information Sciences, Fukui University of Technology, 3-6-1 Gakuen, Fukui 910-8505, Japan cDepartment of Nutritional Science, Faculty of Applied BioScience, Tokyo University of Agriculture, Tokyo 165-8502, Japan. *To whom correspondence should be addressed: Tel.: +81-3-5477-2679; E-mail: [email protected]. Supplementary Fig. S1. Fig. S1. Disruption of PpAOS1 and PpAOS2 genes in P. p a te ns . A, Genomic structures of PpAOS1 and PpAOS2 in the wild-type and targeted PpAOS1 and PpAOS2 knock-out mutants (A5, A19, and A22). The npt II and aph IV expression cassettes were inserted in A5, A19, and A22 strains. B, Genomic PCR data of wild-type, and A5, A19 and A22 strains. P. patens genomic DNA was isolated from protonemata by the CTAB method (Nishiyama et al., Ref. 32). PCR was performed in 50 µL of a reaction mixture containing 1 µL of genomic DNA solution, 1.5 µL of each primer (5 µM), 25 µL of KOD One PCR Master Mix (Toyobo, Japan), and 21 µL of Milli-Q water. PCR was conducted with the following conditions: 30 cycles of 98°C for 10 s, 58°C for 5 s, and 68°C for 15 s. -
Allostery and Cooperativity in Multimeric Proteins: Bond-To-Bond Propensities in Atcase
Allostery and cooperativity in multimeric proteins: bond-to-bond propensities in ATCase Maxwell Hodges1,3, Mauricio Barahona2,3, and Sophia N. Yaliraki1,3 1Department of Chemistry, Imperial College London, South Kensington Campus, London SW7 2AZ, United Kingdom 2Department of Mathematics, Imperial College London, South Kensington Campus, London SW7 2AZ, United Kingdom 3Institute of Chemical Biology, Imperial College London, South Kensington Campus, London SW7 2AZ, United Kingdom September 30, 2019 Abstract Aspartate carbamoyltransferase (ATCase) is a large dodecameric enzyme with six active sites that exhibits allostery: its catalytic rate is modulated by the binding of various substrates at distal points from the active sites. A recently developed method, bond-to-bond propensity analysis, has proven capable of predicting allosteric sites in a wide range of proteins using an energy-weighted atomistic graph obtained from the protein structure and given knowledge only of the location of the active site. Bond-to-bond propensity establishes if energy fluctuations at given bonds have significant effects on any other bond in the protein, by considering their propagation through the protein graph. In this work, we use bond-to-bond propensity analysis to study different aspects of ATCase activity using three different protein structures and sources of fluctuations. First, we predict key residues and bonds involved in the transition between inactive (T) and active (R) states of ATCase by analysing allosteric substrate binding as a source of energy perturbations in the protein graph. Our computational results also indicate that the effect of multiple allosteric binding is non linear: a switching effect is observed after a particular number and arrangement of substrates is bound suggesting a form of long range communication between the distantly arranged allosteric sites. -
Glycolysis Citric Acid Cycle Oxidative Phosphorylation Calvin Cycle Light
Stage 3: RuBP regeneration Glycolysis Ribulose 5- Light-Dependent Reaction (Cytosol) phosphate 3 ATP + C6H12O6 + 2 NAD + 2 ADP + 2 Pi 3 ADP + 3 Pi + + 1 GA3P 6 NADP + H Pi NADPH + ADP + Pi ATP 2 C3H4O3 + 2 NADH + 2 H + 2 ATP + 2 H2O 3 CO2 Stage 1: ATP investment ½ glucose + + Glucose 2 H2O 4H + O2 2H Ferredoxin ATP Glyceraldehyde 3- Ribulose 1,5- Light Light Fx iron-sulfur Sakai-Kawada, F Hexokinase phosphate bisphosphate - 4e + center 2016 ADP Calvin Cycle 2H Stroma Mn-Ca cluster + 6 NADP + Light-Independent Reaction Phylloquinone Glucose 6-phosphate + 6 H + 6 Pi Thylakoid Tyr (Stroma) z Fe-S Cyt f Stage 1: carbon membrane Phosphoglucose 6 NADPH P680 P680* PQH fixation 2 Plastocyanin P700 P700* D-(+)-Glucose isomerase Cyt b6 1,3- Pheophytin PQA PQB Fructose 6-phosphate Bisphosphoglycerate ATP Lumen Phosphofructokinase-1 3-Phosphoglycerate ADP Photosystem II P680 2H+ Photosystem I P700 Stage 2: 3-PGA Photosynthesis Fructose 1,6-bisphosphate reduction 2H+ 6 ADP 6 ATP 6 CO2 + 6 H2O C6H12O6 + 6 O2 H+ + 6 Pi Cytochrome b6f Aldolase Plastoquinol-plastocyanin ATP synthase NADH reductase Triose phosphate + + + CO2 + H NAD + CoA-SH isomerase α-Ketoglutarate + Stage 2: 6-carbonTwo 3- NAD+ NADH + H + CO2 Glyceraldehyde 3-phosphate Dihydroxyacetone phosphate carbons Isocitrate α-Ketoglutarate dehydogenase dehydrogenase Glyceraldehyde + Pi + NAD Isocitrate complex 3-phosphate Succinyl CoA Oxidative Phosphorylation dehydrogenase NADH + H+ Electron Transport Chain GDP + Pi 1,3-Bisphosphoglycerate H+ Succinyl CoA GTP + CoA-SH Aconitase synthetase -
How to Build a Water-Splitting Machine: Structural Insights Into Photosystem II Assembly
bioRxiv preprint doi: https://doi.org/10.1101/2020.09.14.294884; this version posted September 15, 2020. The copyright holder for this preprint (which was not certified by peer review) is the author/funder. All rights reserved. No reuse allowed without permission. How to build a water-splitting machine: structural insights into photosystem II assembly Jure Zabret1#, Stefan Bohn2#, Sandra K. Schuller3,4, Oliver Arnolds5, Madeline Möller1, Jakob Meier-Credo6, Pasqual Liauw1, Aaron Chan7, Emad Tajkhorshid7, Julian D. Langer6,8, Raphael Stoll5, Anja Krieger-Liszkay9, Benjamin D. Engel2,10,11, Till Rudack12,13,*, Jan M. Schuller3,4,*, Marc M. Nowaczyk1,* Affiliations: 1Department of Plant Biochemistry, Faculty of Biology & Biotechnology, Ruhr University Bochum, 44780 Bochum, Germany. 2Department of Molecular Structural Biology, Max Planck Institute of Biochemistry, 82152 Martinsried, Germany. 3Department of Structural Cell Biology, Max Planck Institute of Biochemistry, 82152 Martinsried, Germany. 4 SYNMIKRO Research Center, Philipps-University Marburg, 35043 Marburg, Germany. 5Biomolecular Spectroscopy and RUBiospek|NMR, Faculty of Chemistry and Biochemistry, Ruhr-University Bochum, 44780 Bochum, Germany 6Department of Molecular Membrane Biology, Max Planck Institute of Biophysics, 60438 Frankfurt/Main, Germany. 7NIH Center for Macromolecular Modeling and Bioinformatics, Beckman Institute for Advanced Science and Technology, Department of Biochemistry, and Center for Biophysics and Quantitative Biology, University of Illinois at Urbana-Champaign, Urbana, Illinois 8Max Planck Institute for Brain Research, Max von Laue Strasse 4, 60438 Frankfurt/Main, Germany. 9Université Paris-Saclay, CEA, CNRS, Institute for Integrative Biology of the Cell (I2BC), 91198, Gif-sur-Yvette, France 10Helmholtz Pioneer Campus, Helmholtz Zentrum München, Ingolstädter Landstraße 1, 85764 Neuherberg, Germany. 11Department of Chemistry, Technical University of Munich, Lichtenbergstraße 4, 85748 Garching, Germany. -
Metabolomics-Assisted Proteomics Identifies Succinylation and SIRT5 As Important Regulators of Cardiac Function
Metabolomics-assisted proteomics identifies succinylation and SIRT5 as important regulators of cardiac function Sushabhan Sadhukhana, Xiaojing Liub,c,d, Dongryeol Ryue, Ornella D. Nelsona, John A. Stupinskif, Zhi Lia, Wei Cheng, Sheng Zhangg, Robert S. Weissf, Jason W. Locasaleb,c,d, Johan Auwerxe,1, and Hening Lina,h,1 aDepartment of Chemistry and Chemical Biology, Cornell University, Ithaca, NY 14853; bDuke Cancer Institute, Duke University School of Medicine, Durham, NC 27710; cDuke Molecular Physiology Institute, Duke University School of Medicine, Durham, NC 27710; dDepartment of Pharmacology and Cancer Biology, Duke University School of Medicine, Durham, NC 27710; eLaboratory of Integrative and Systems Physiology, School of Life Sciences, École Polytechnique Fédérale de Lausanne, 1015 Lausanne, Switzerland; fDepartment of Biomedical Sciences, Cornell University, Ithaca, NY 14853; gProteomics & Mass Spectrometry Facility, Institute of Biotechnology, Cornell University, Ithaca, NY 14853; and hHoward Hughes Medical Institute, Cornell University, Ithaca, NY 14853 Edited by Kevan M. Shokat, University of California, San Francisco, CA, and approved March 9, 2016 (received for review October 7, 2015) Cellular metabolites, such as acyl-CoA, can modify proteins, leading SIRT4 and SIRT5 have very weak deacetylase activities (14). SIRT5 to protein posttranslational modifications (PTMs). One such PTM is possesses unique enzymatic activity on hydrolyzing negatively lysine succinylation, which is regulated by sirtuin 5 (SIRT5). Although charged