Identification and Characterization of a Single-Stranded DNA-Binding Protein from the Archaeon Methanococcus Jannaschii
Total Page:16
File Type:pdf, Size:1020Kb
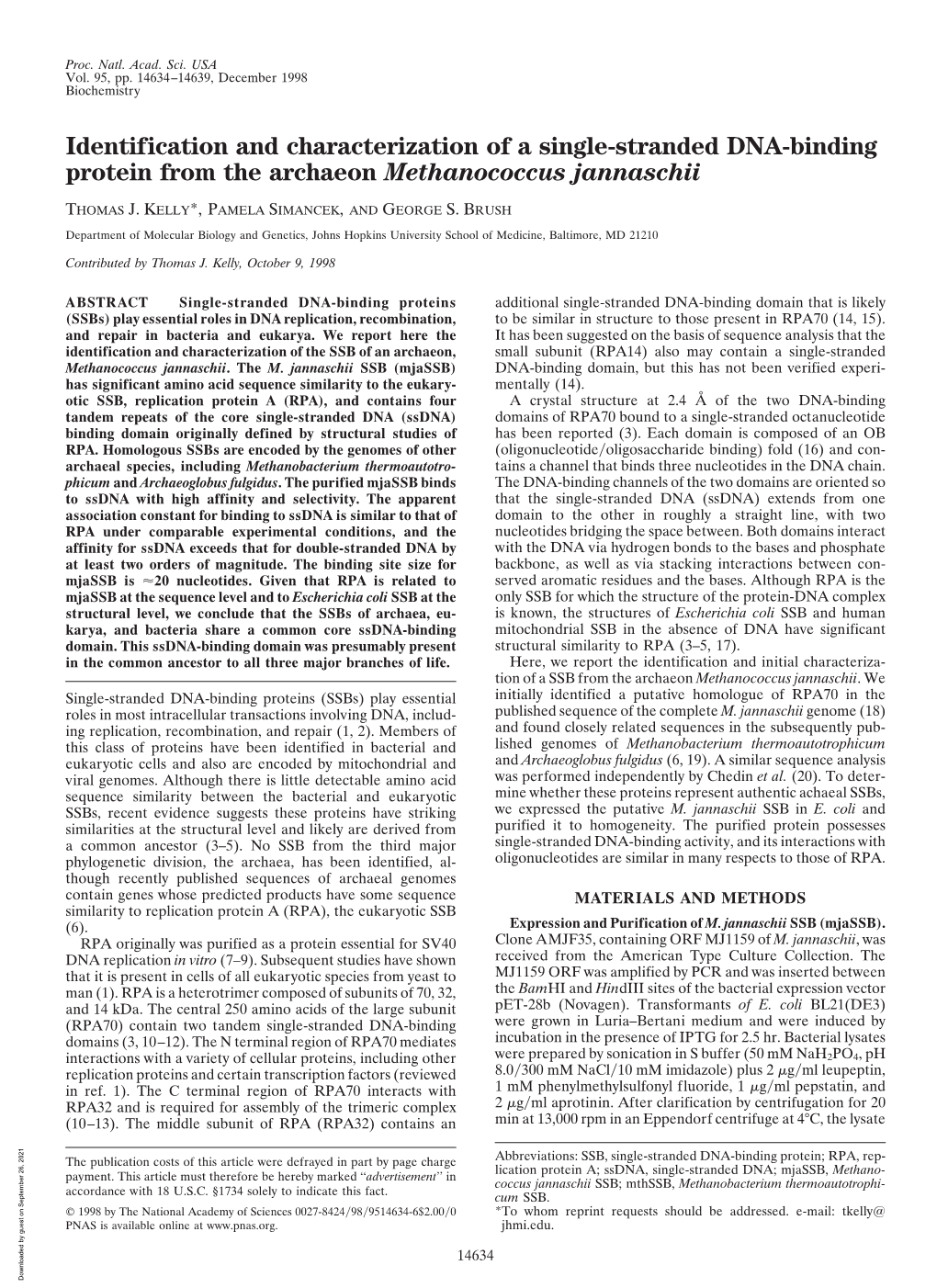
Load more
Recommended publications
-
Transcription-Replication Collisions—A Series of Unfortunate Events
biomolecules Review Transcription-Replication Collisions—A Series of Unfortunate Events Commodore St Germain 1,2,*, Hongchang Zhao 2 and Jacqueline H. Barlow 2,* 1 School of Mathematics and Science, Solano Community College, 4000 Suisun Valley Road, Fairfield, CA 94534, USA 2 Department of Microbiology and Molecular Genetics, University of California Davis, One Shields Avenue, Davis, CA 95616, USA; [email protected] * Correspondence: [email protected] (C.S.G.); [email protected] (J.H.B.) Abstract: Transcription-replication interactions occur when DNA replication encounters genomic regions undergoing transcription. Both replication and transcription are essential for life and use the same DNA template making conflicts unavoidable. R-loops, DNA supercoiling, DNA secondary structure, and chromatin-binding proteins are all potential obstacles for processive replication or transcription and pose an even more potent threat to genome integrity when these processes co-occur. It is critical to maintaining high fidelity and processivity of transcription and replication while navigating through a complex chromatin environment, highlighting the importance of defining cellular pathways regulating transcription-replication interaction formation, evasion, and resolution. Here we discuss how transcription influences replication fork stability, and the safeguards that have evolved to navigate transcription-replication interactions and maintain genome integrity in mammalian cells. Keywords: DNA replication; transcription; R-loops; replication stress Citation: St Germain, C.; Zhao, H.; Barlow, J.H. Transcription-Replication Collisions—A Series of Unfortunate Events. Biomolecules 2021, 11, 1249. 1. Introduction https://doi.org/10.3390/ From bacteria to humans, transcription has been identified as a source of genome biom11081249 instability, initially observed as spontaneous recombination referred to as transcription- associated recombination (TAR) [1]. -
CHK1 Inhibition Is Synthetically Lethal with Loss of B-Family DNA Polymerase Function in Human Lung and Colorectal Cancer Cells
Author Manuscript Published OnlineFirst on March 11, 2020; DOI: 10.1158/0008-5472.CAN-19-1372 Author manuscripts have been peer reviewed and accepted for publication but have not yet been edited. 1 Title: CHK1 inhibition is synthetically lethal with loss of B-family DNA polymerase function in human lung and colorectal cancer cells. Author List: Rebecca F. Rogers1, Mike I. Walton1, Daniel L. Cherry2, Ian Collins1, Paul A. Clarke1, Michelle D. Garrett2* and Paul Workman1* Affiliations: 1Cancer Research UK Cancer Therapeutics Unit, The Institute of Cancer Research, London, SM2 5NG, UK 2 School of Biosciences, Stacey Building, University of Kent, Canterbury, Kent, CT2 7NJ, UK Running title: Synthetic lethality of CHK1 and DNA polymerase inhibition *Corresponding authors: Michelle D Garrett and Paul Workman Corresponding Author Information Michelle D Garrett School of Biosciences, Stacey Building, University of Kent, Canterbury, Kent, CT2 7NJ, UK. Email: [email protected] Phone: +44 (0)1227 816140 Paul Workman Cancer Research UK Cancer Therapeutics Unit, The Institute of Cancer Research, London, SM2 5NG, UK. Email: [email protected] Phone: +44 (0)20 7153 5209 Conflict of interest statement: IC, MDG, MIW, RFR, PC and PW are current or past employees of The Institute of Cancer Research, which has a commercial interest in the discovery and development of CHK1 inhibitors, including SRA737, and operates a rewards-to- inventors scheme. IC, MDG and MIW have been involved in a commercial collaboration on CHK1 inhibitors with Sareum Ltd and intellectual property arising from the program, including SRA737, was licensed to Sierra Oncology. IC is a consultant for Sierra Oncology, Adorx Ltd, Epidarex LLP and Enterprise Therapeutics Ltd and holds equity in Monte Rosa Therapeutics AG. -
Expression of an Ortholog of Replication Protein A1 (RPA1)
Proc. Natl. Acad. Sci. USA Vol. 94, pp. 9979–9983, September 1997 Plant Biology Expression of an ortholog of replication protein A1 (RPA1) is induced by gibberellin in deepwater rice (cell divisionyintercalary meristemyinternodal growthyOryza sativa) ESTHER VAN DER KNAAP*, SANDRINE JAGOUEIX*†, AND HANS KENDE‡ Michigan State University–Department of Energy Plant Research Laboratory, Michigan State University, East Lansing, MI 48824-1312 Contributed by Hans Kende, July 16, 1997 ABSTRACT Internodes of deepwater rice are induced to antagonist of GA action in rice (6). Growth of the internode grow rapidly when plants become submerged. This adaptation is, ultimately, promoted by GA (5). enables deepwater rice to keep part of its foliage above the The primary target tissue of GA is the intercalary meristem rising flood waters during the monsoon season and to avoid of the internode, where GA enhances cell division activity and drowning. This growth response is, ultimately, elicited by the cell elongation (5, 7, 8). The intercalary meristem is a zone of plant hormone gibberellin (GA). The primary target tissue for about 3 mm in length and is located at the base of the internode GA action is the intercalary meristem of the internode. Using (9). Cells are displaced from the intercalary meristem into the differential display of mRNA, we have isolated a number of elongation zone, where they reach their final size. In GA- genes whose expression in the intercalary meristem is regu- treated internodes, the final cell length is about three to four lated by GA. The product of one of these genes was identified times longer than in control internodes (5, 7). -
Monitoring Replication Protein a (RPA) Dynamics in Homologous
Marquette University e-Publications@Marquette Biological Sciences Faculty Research and Biological Sciences, Department of Publications 9-19-2017 Monitoring Replication Protein A (RPA) Dynamics in Homologous Recombination Through Site-specific ncorI poration of Non- canonical Amino Acids Nilisha Pokhrel Marquette University Sofia Origanti Marquette University, [email protected] Eric Parker Davenport Marquette University Disha M. Gandhi Marquette University Kyle Kaniecki Columbia University See next page for additional authors Published version. Nucleic Acids Research, Vol. 45, No. 16 (September 19, 2017): 9413-9426. DOI. Authors Nilisha Pokhrel, Sofia Origanti, Eric Parker Davenport, Disha M. Gandhi, Kyle Kaniecki, Ryan A. Mehl, Eric C. Greene, Chris Dockendorff, and Edwin Antony This article is available at e-Publications@Marquette: https://epublications.marquette.edu/bio_fac/603 Published online 12 July 2017 Nucleic Acids Research, 2017, Vol. 45, No. 16 9413–9426 doi: 10.1093/nar/gkx598 Monitoring Replication Protein A (RPA) dynamics in homologous recombination through site-specific incorporation of non-canonical amino acids Nilisha Pokhrel1, Sofia Origanti1, Eric Parker Davenport1, Disha Gandhi2, Kyle Kaniecki3,4, Ryan A. Mehl5, Eric C. Greene3, Chris Dockendorff2 and Edwin Antony1,* 1Department of Biological Sciences, Marquette University, Milwaukee, WI 53201, USA, 2Department of Chemistry, Marquette University, Milwaukee, WI 53201, USA, 3Department of Biochemistry and Molecular Biophysics, Columbia University, New York, NY -
DNA Unwinding by Replication Protein a Is a Property of the 32 Kda Subunit
1993 Oxford University Press Nucleic Acids Research, 1993, Vol. 21, No. 16 3659-3665 DNA unwinding by replication protein A is a property of the 70 kDa subunit and is facilitated by phosphorylation of the 32 kDa subunit Anthi Georgaki and Ulrich Hubscher* Department of Veterinary Biochemistry, University of Zurich-lrchel, Winterthurerstrasse 190, CH-8057 Zurich, Switzerland Received May 28, 1993; Revised and Accepted July 1, 1993 ABSTRACT Replication protein A (RP-A) is a heterotrimeric single- three subunits of 70 kDa, 32-34 kDa and 11-14 kDa and binds stranded DNA binding protein with important functions preferentially to ssDNA through its 70 kDa subunit. RP-A in DNA replication, DNA repair and DNA recombination. appears to have an important role in the T antigen dependent We have found that RP-A from calf thymus can unwind generation of a ss region at the SV40 origin prior to initiation DNA in the absence of ATP and MgCI2, two essential of DNA synthesis. This protein is highly conserved since it has cofactors for bona fide DNA helicases (Georgaki, A., been found to have a similar subunit structure and function in Strack, B., Podust, V. and Hubscher, U. FEBS Lett. 308, Saccharomyces cerevisiae [3]. RP-A has a preference to bind 240 - 244, 1992). DNA unwinding by RP-A was found to ss pyrimidine stretches in a stoichiometry of 1 molecule to to be sensitive to MgCI2, ATP, heating and freezing/ 30 bases [2]. In addition, it has direct effects on DNA thawing. Escherichia coil single stranded DNA binding polymerases a and a [4]. -
Replication Protein a Is an Independent Prognostic Indicator with Potential Therapeutic Implications in Colon Cancer
Modern Pathology (2007) 20, 159–166 & 2007 USCAP, Inc All rights reserved 0893-3952/07 $30.00 www.modernpathology.org Replication protein A is an independent prognostic indicator with potential therapeutic implications in colon cancer Nikolaos Givalos1, Hariklia Gakiopoulou2, Melina Skliri2, Katerina Bousboukea2, Anastasia E Konstantinidou2, Penelope Korkolopoulou2, Maria Lelouda2, Gregory Kouraklis1, Efstratios Patsouris2 and Gabriel Karatzas1 1Department of Surgery, Medical School, National Kapodistrian University of Athens, Athens, Greece and 2Department of Pathology, Medical School, National Kapodistrian University of Athens, Athens, Greece Replication protein A (RPA), a component of the origin recognition complex, is required for stabilization of single-stranded DNA at early and later stages of DNA replication being thus critical for eukaryotic DNA replication. Experimental studies in colon cancer cell lines have shown that RPA protein may be the target of cytotoxins designed to inhibit cellular proliferation. This is the first study to investigate the expression of RPA1 and RPA2 subunits of RPA protein and assess their prognostic value in colon cancer patients. We analyzed immunohistochemically the expression of RPA1 and RPA2 proteins in a series of 130 colon cancer resection specimens in relation to conventional clinicopathological parameters and patients’ survival. Statistical significant positive associations emerged between: (a) RPA1 and RPA2 protein expressions (P ¼ 0.0001), (b) RPA1 and RPA2 labelling indices (LIs) and advanced stage of the disease (P ¼ 0.001 and 0.003, respectively), (c) RPA1 and RPA2 LIs and the presence of lymph node metastasis (P ¼ 0.002 and 0.004, respectively), (d) RPA1 LI and the number of infiltrated lymph nodes (P ¼ 0.021), (e) RPA2 LI and histological grade of carcinomas (P ¼ 0.05). -
Congenital Diseases of DNA Replication: Clinical Phenotypes and Molecular Mechanisms
International Journal of Molecular Sciences Review Congenital Diseases of DNA Replication: Clinical Phenotypes and Molecular Mechanisms Megan Schmit and Anja-Katrin Bielinsky * Department of Biochemistry, Molecular Biology, and Biophysics, University of Minnesota, Minneapolis, MN 55455, USA; [email protected] * Correspondence: [email protected] Abstract: Deoxyribonucleic acid (DNA) replication can be divided into three major steps: initiation, elongation and termination. Each time a human cell divides, these steps must be reiteratively carried out. Disruption of DNA replication can lead to genomic instability, with the accumulation of point mutations or larger chromosomal anomalies such as rearrangements. While cancer is the most common class of disease associated with genomic instability, several congenital diseases with dysfunctional DNA replication give rise to similar DNA alterations. In this review, we discuss all congenital diseases that arise from pathogenic variants in essential replication genes across the spectrum of aberrant replisome assembly, origin activation and DNA synthesis. For each of these conditions, we describe their clinical phenotypes as well as molecular studies aimed at determining the functional mechanisms of disease, including the assessment of genomic stability. By comparing and contrasting these diseases, we hope to illuminate how the disruption of DNA replication at distinct steps affects human health in a surprisingly cell-type-specific manner. Keywords: Meier-Gorlin syndrome; natural killer cell deficiency; X-linked pigmentary reticulate disorder; Van Esch-O’Driscoll disease; IMAGe syndrome; FILS syndrome; Rothmund-Thomson syndrome; Baller-Gerold syndrome; RAPADILINO Citation: Schmit, M.; Bielinsky, A.-K. Congenital Diseases of DNA Replication: Clinical Phenotypes and 1. Introduction Molecular Mechanisms. Int. J. Mol. 1.1. Replication Initiation Sci. -
Switch-Like Control of Helicase Processivity by Single-Stranded DNA
bioRxiv preprint doi: https://doi.org/10.1101/2020.07.09.194779; this version posted July 9, 2020. The copyright holder for this preprint (which was not certified by peer review) is the author/funder, who has granted bioRxiv a license to display the preprint in perpetuity. It is made available under aCC-BY 4.0 International license. 1 Switch-like control of helicase processivity by single-stranded DNA 2 binding protein 3 4 Barbara Stekas1, Masayoshi Honda2, Maria Spies2, Yann R. Chemla1,3,* 5 1Department of Physics, University of Illinois, Urbana-Champaign, Urbana 6 2Department of Biochemistry, Carver College of Medicine, University of Iowa, Iowa City 7 3Center for the Physics of Living Cells, University of Illinois, Urbana-Champaign, Urbana 8 9 Helicases utilize the energy of NTP hydrolysis to translocate along single-stranded nucleic acids (NA) and 10 unwind the duplex. In the cell, helicases function in the context of other NA-associated proteins which 11 regulate helicase function. For example, single-stranded DNA binding proteins are known to enhance 12 helicase activity, although the underlying mechanisms remain largely unknown. F. acidarmanus XPD 13 helicase serves as a model for understanding the molecular mechanisms of Superfamily 2B helicases, and 14 previous work has shown that its activity is enhanced by the cognate single-stranded DNA binding protein 15 RPA2. Here, single-molecule optical trap measurements of the unwinding activity of a single XPD helicase 16 in the presence of RPA2 reveal a mechanism in which XPD interconverts between two states with different 17 processivities and transient RPA2 interactions stabilize the more processive state, activating a latent 18 “processivity switch” in XPD. -
Colon Cancer-Associated Mutator DNA Polymerase Δ Variant Causes
Colon cancer-associated mutator DNA polymerase δ PNAS PLUS variant causes expansion of dNTP pools increasing its own infidelity Tony M. Mertza, Sushma Sharmab, Andrei Chabesb,c, and Polina V. Shcherbakovaa,1 aEppley Institute for Research in Cancer and Allied Diseases, University of Nebraska Medical Center, Omaha, NE 68198; and bDepartment of Medical Biochemistry and Biophysics and cLaboratory for Molecular Infection Medicine Sweden, Umeå University, SE 90187 Umeå, Sweden Edited by Lawrence A. Loeb, University of Washington School of Medicine, Seattle, WA, and accepted by the Editorial Board March 2, 2015 (received for review December 3, 2014) Defects in DNA polymerases δ (Polδ) and e (Pole) cause hereditary main mutations were modeled in yeast and found to increase colorectal cancer and have been implicated in the etiology of some mutation rate, supporting their role in the development of sporadic colorectal and endometrial tumors. We previously re- hypermutated tumors (19, 24). Because of these discoveries, ported that the yeast pol3-R696W allele mimicking a human recent publications emphasized proofreading deficiency as the cancer-associated variant, POLD1-R689W, causes a catastrophic in- initiating cause of some human tumors (25–28). The potential crease in spontaneous mutagenesis. Here, we describe the mech- role of base selectivity defects in human cancers received much anism of this extraordinary mutator effect. We found that the less attention. At the same time, evidence is accumulating that base selectivity defects do occur in tumors and can have dramatic mutation rate increased synergistically when the R696W mutation e was combined with defects in Polδ proofreading or mismatch re- consequences for genome stability. -
Replication Protein a Presents Canonical Functions and Is Also Involved in the Differentiation Capacity of Trypanosoma Cruzi
RESEARCH ARTICLE Replication Protein A Presents Canonical Functions and Is Also Involved in the Differentiation Capacity of Trypanosoma cruzi Raphael Souza Pavani1,2, Marcelo Santos da Silva1,2, Carlos Alexandre Henrique Fernandes3, Flavia Souza Morini4, Christiane Bezerra Araujo1,2, Marcos Roberto de Mattos Fontes3, Osvaldo Augusto Sant'Anna2,5, Carlos Renato Machado6, Maria Isabel Cano7, Stenio Perdigão Fragoso4, Maria Carolina Elias1,2* 1 LaboratoÂrio Especial de Ciclo Celular, Instituto Butantan, São Paulo, São Paulo, Brazil, 2 Center of Toxins, Immune Response and Cell SignalingÐCeTICS, Instituto Butantan, São Paulo, São Paulo, Brazil, a11111 3 Departamento de FõÂsica e BiofõÂsica, Instituto de Biociências, Universidade Estadual Paulista JuÂlio de Mesquita Filho -UNESP, Botucatu, São Paulo, Brazil, 4 Instituto Carlos Chagas, Fiocruz-PR, Brazil, 5 LaboratoÂrio de ImunoquõÂmica, Instituto Butantan, São Paulo, São Paulo, Brazil, 6 Departamento de BioquõÂmica e Imunologia, ICB, Universidade Federal de Minas Gerais, Belo Horizonte, Minas Gerais, Brazil, 7 Departamento de GeneÂtica, Instituto de Biociências, Universidade Estadual Paulista Julio Mesquita FilhoÐ UNESP, Botucatu, São Paulo, Brazil * [email protected] OPEN ACCESS Citation: Pavani RS, da Silva MS, Fernandes CAH, Abstract Morini FS, Araujo CB, Fontes MRdM, et al. (2016) Replication Protein A Presents Canonical Functions Replication Protein A (RPA), the major single stranded DNA binding protein in eukaryotes, and Is Also Involved in the Differentiation Capacity of Trypanosoma cruzi. PLoS Negl Trop Dis 10(12): is composed of three subunits and is a fundamental player in DNA metabolism, participating e0005181. doi:10.1371/journal.pntd.0005181 in replication, transcription, repair, and the DNA damage response. In human pathogenic Editor: Carlos A. -
Functional Diversification of Replication Protein A
| INVESTIGATION Functional Diversification of Replication Protein A Paralogs and Telomere Length Maintenance in Arabidopsis Behailu B. Aklilu,* François Peurois,†,‡ Carole Saintomé,†,‡ Kevin M. Culligan,§ Daniela Kobbe,** Catherine Leasure,* Michael Chung,* Morgan Cattoor,* Ryan Lynch,* Lauren Sampson,* John Fatora,* and Dorothy E. Shippen* *Department of Biochemistry and Biophysics, Texas A&M University, College Station, Texas 77843-2128, †Sorbonne Université, UFR927, 4 place Jussieu, 75252, Paris Cedex 05, France, ‡Structure et Instabilité des Génomes, INSERM U1154/CNRS UMR 7196, Muséum National d’Histoire Naturelle, Paris 75005, France, §Department of Molecular, Cellular and Biomedical Sciences, University of New Hampshire, Durham, New Hampshire 03824, and **Botanical Institute II, Karlsruhe Institute of Technology (KIT), Karlsruhe 76131, Germany ORCID IDs: 0000-0001-9821-5154 (B.B.A.); 0000-0003-0562-2047 (D.E.S.) ABSTRACT Replication protein A (RPA) is essential for many facets of DNA metabolism. The RPA gene family expanded in Arabidopsis thaliana with five phylogenetically distinct RPA1 subunits (RPA1A-E), two RPA2 (RPA2A and B), and two RPA3 (RPA3A and B). RPA1 paralogs exhibit partial redundancy and functional specialization in DNA replication (RPA1B and RPA1D), repair (RPA1C and RPA1E), and meiotic recombination (RPA1A and RPA1C). Here, we show that RPA subunits also differentially impact telomere length set point. Loss of RPA1 resets bulk telomeres at a shorter length, with a functional hierarchy for replication group over repair and meiosis group RPA1 subunits. Plants lacking RPA2A, but not RPA2B, harbor short telomeres similar to the replication group. Telomere shortening does not correlate with decreased telomerase activity or deprotection of chromosome ends in rpa mutants. -
RNA–DNA Hybrids: Double-Edged Swords
RESEARCH HIGHLIGHTS Nature Reviews Genetics | Published online 21 Nov 2016; doi:10.1038/nrg.2016.153 Henry Steadman/Photodisc/Getty DNA REPAIR RNA–DNA hybrids: double-edged swords RNA–DNA hybrids are associated with DNA from the DSB in both directions and subsequent damage and genome instability and are thus transcript–template hybridization. typically thought of as harmful. A paper in Cell HR-mediated DSB repair involves resection of now reports that RNA–DNA hybrids have a the 5′ strand on either side of the DSB, which functional role in the homologous recombination leaves long single-stranded DNA (ssDNA) (HR)-mediated repair of double strand DNA 3′ overhangs. These ssDNA stretches are bound breaks (DSBs) and serve to protect genome and protected by replication protein A (RPA) integrity in yeast. before completion of the repair process, which Ohle et al. sought to better understand the occurs through invasion of homologous DNA on function of RNase H enzymes, which are known the sister chromatid. The authors reasoned that to degrade RNA–DNA hybrids. To this end, they the ssDNA overhangs could serve as a template generated RNase H deletion mutants of for the transcription generating RNA–DNA Schizosaccharomyces pombe and analysed their hybrids around DSBs. responses to DNA damage. Strains lacking RNase Consistent with this model, induction of DSBs H were highly sensitive to drug-induced DSBs strongly increased RNA polymerase II (Pol II) and contained increased levels of RNA–DNA levels around the cleavage site, indicative of hybrids. To enable further investigation, the active transcription at DSBs. In addition, the authors engineered a system that allowed them recruitment of RPA was strongly impaired in to induce a chromosomal DSB at a specific cells lacking RNase H and increased in cells cleavage site.