The `Humped' Soil Production Function: Eroding Arnhem Land
Total Page:16
File Type:pdf, Size:1020Kb
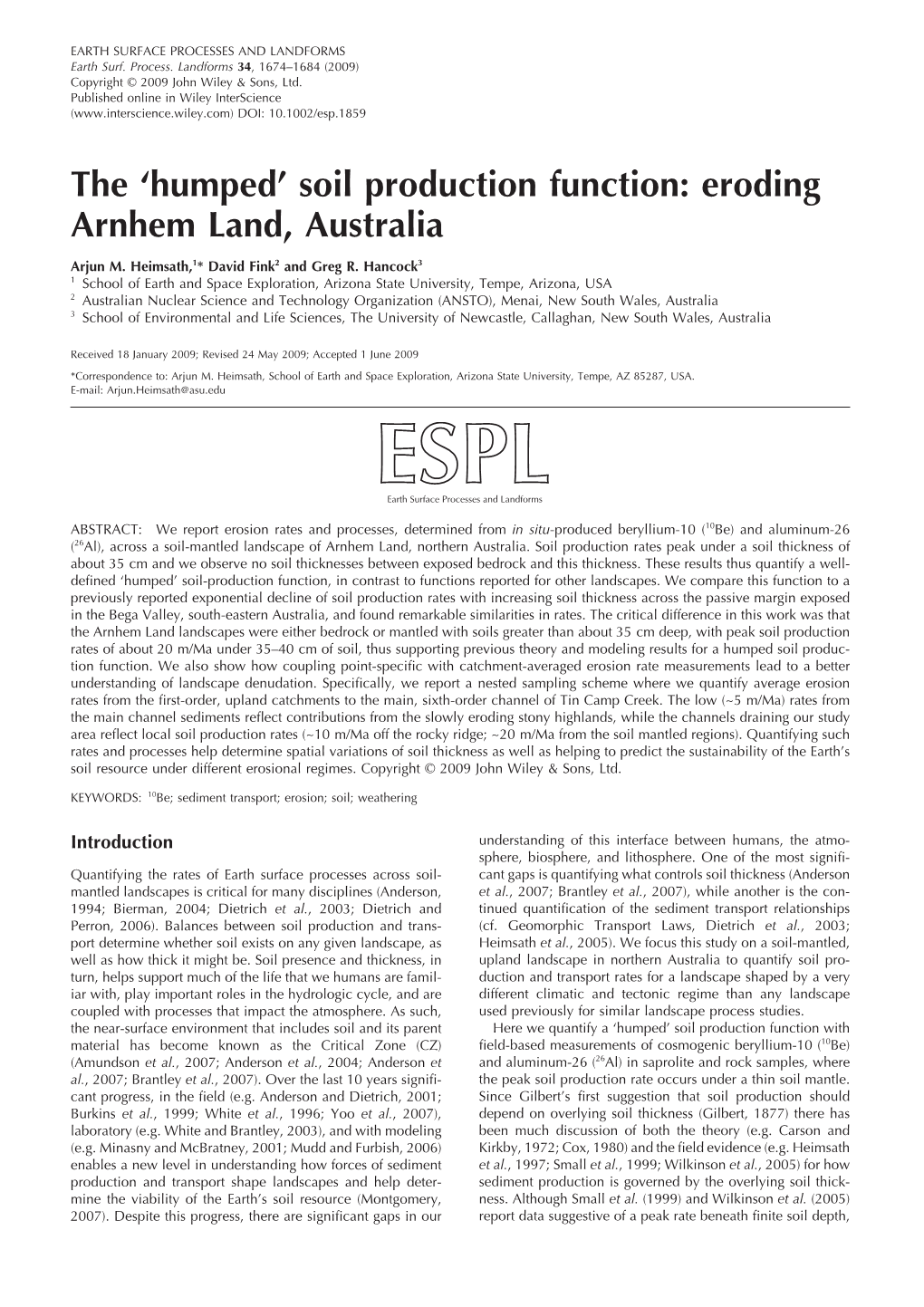
Load more
Recommended publications
-
The Effect of Time on Soil Development: Study of a Soil Chronosequence from Reunion Island, Indian Ocean
University of Wollongong Research Online Faculty of Science, Medicine & Health - Honours Theses University of Wollongong Thesis Collections 2016 The effect of time on soil development: study of a soil chronosequence from Reunion Island, Indian Ocean Alex Hannan-Joyner Follow this and additional works at: https://ro.uow.edu.au/thsci University of Wollongong Copyright Warning You may print or download ONE copy of this document for the purpose of your own research or study. The University does not authorise you to copy, communicate or otherwise make available electronically to any other person any copyright material contained on this site. You are reminded of the following: This work is copyright. Apart from any use permitted under the Copyright Act 1968, no part of this work may be reproduced by any process, nor may any other exclusive right be exercised, without the permission of the author. Copyright owners are entitled to take legal action against persons who infringe their copyright. A reproduction of material that is protected by copyright may be a copyright infringement. A court may impose penalties and award damages in relation to offences and infringements relating to copyright material. Higher penalties may apply, and higher damages may be awarded, for offences and infringements involving the conversion of material into digital or electronic form. Unless otherwise indicated, the views expressed in this thesis are those of the author and do not necessarily represent the views of the University of Wollongong. Recommended Citation Hannan-Joyner, Alex, The effect of time on soil development: study of a soil chronosequence from Reunion Island, Indian Ocean, BSci Hons, School of Earth & Environmental Sciences, University of Wollongong, 2016. -
Biomechanical and Biochemical Effects Recorded in the Tree Root Zone – Soil Memory, Historical Contingency and Soil Evolution Under Trees
Plant Soil (2018) 426:109–134 https://doi.org/10.1007/s11104-018-3622-9 REGULAR ARTICLE Biomechanical and biochemical effects recorded in the tree root zone – soil memory, historical contingency and soil evolution under trees Łukasz Pawlik & Pavel Šamonil Received: 17 September 2017 /Accepted: 1 March 2018 /Published online: 15 March 2018 # The Author(s) 2018 Abstract increase in soil spatial complexity. We hypothesized that Background and aims The changing soils is a never- trees can be a strong local factor intensifying, blocking ending process moderated by numerous biotic and abi- or modifying pedogenetic processes, leading to local otic factors. Among these factors, trees may play a changes in soil complexity (convergence, divergence, critical role in forested landscapes by having a large or polygenesis). These changes are hypothetically con- imprint on soil texture and chemical properties. During trolled by regionally predominating soil formation their evolution, soils can follow convergent or divergent processes. development pathways, leading to a decrease or an Methods To test the main hypothesis, we described the pedomorphological features of soils under tree stumps of fir, beech and hemlock in three soil regions: Haplic Highlights Cambisols (Turbacz Reserve, Poland), Entic Podzols 1) The architecture of tree root systems controls soil physical and (Žofínský Prales Reserve, Czech Republic) and Albic chemical properties. Podzols (Upper Peninsula, Michigan, USA). Soil pro- 2) The predominating pedogenetic process significantly modifies files under the stumps, as well as control profiles on sites the effect of trees on soil. 3) Trees are a factor in polygenesis in Haplic Cambisols at the currently not occupied by trees, were analyzed in the pedon scale. -
Deficit Saline Water Irrigation Under Reduced Tillage and Residue Mulch
www.nature.com/scientificreports OPEN Defcit saline water irrigation under reduced tillage and residue mulch improves soil health in sorghum‑wheat cropping system in semi‑arid region Pooja Gupta Soni1,2, Nirmalendu Basak 1*, Arvind Kumar Rai 1*, Parul Sundha1, Bhaskar Narjary1, Parveen Kumar1, Gajender Yadav1, Satyendra Kumar1 & Rajender Kumar Yadav1 Judicious application of saline water except for critical growth stages, could be the only practical solution to meet the crop water demand in arid and semi‑arid regions, due to limited access to freshwater, especially during dry winter months. A feld experiment was conducted to study the efect of tillage [conventional (CT), reduced (RT), and zero (ZT)], rice straw mulch and defcit saline‑water irrigation in wheat (100, 80 and 60% of wheat water requirement, CWR) followed by rainfed sorghum on soil properties and the yields of the cropping system. Yields of both the crops were comparable between RT and CT, but the wheat yield was reduced in ZT. The RT, mulching and defcit saline irrigation in wheat season (60% CWR) increased the sorghum fodder yield. Olsen’s P (8.7–20.6%) and NH4OAc‑K (2.5–7.5%) increased in RT and ZT, respectively, over CT under both the crops. Defcit −1 irrigation reduced soil salinity (ECe) by 0.73–1.19 dS m after each crop cycle, while soil microbial biomass C (MBC) and N (MBN), dehydrogenase, urease and alkaline phosphatase reduced with an increase in ECe. The α‑glucosidase, MBC, ECe, KMnO4oxidizable N, and urease were identifed as major contributors in developing the soil health index. -
Towards a Prediction of Landscape Evolution from Chemical Weathering and Soil Production
TOWARDS A PREDICTION OF LANDSCAPE EVOLUTION FROM CHEMICAL WEATHERING AND SOIL PRODUCTION A thesis submitted in partial fulfillment of the requirements for the degree of Master of Science By ERIC ALAN JACKSON B.S., Wright State University, 2013 2017 Wright State University WRIGHT STATE UNIVERSITY GRADUATE SCHOOL December 15, 2017 I HEREBY RECOMMEND THAT THE THESIS PREPARED UNDER MY SUPERVISION BY Eric Alan Jackson ENTITLED Towards a Prediction of Landscape Evolution from Chemical Weathering and Soil Production BE ACCEPTED IN PARTIAL FULFLLMENT OF THE REQUIREMENTS FOR THE DEGREE OF Master of Science. __________________________ Allen Hunt, Ph.D. Thesis Director __________________________ Jason Deibel, Ph.D. Chair, Department of Physics Committee on Final Examination _________________________ Thomas Skinner, Ph.D. _________________________ Jerry Clark, Ph.D. _________________________ Barry Milligan, Ph.D. Interim Dean of the Graduate School ABSTRACT Jackson, Eric Alan. M.S. Department of Physics, Wright State University, 2017. Towards a Prediction of Landscape Evolution from Chemical Weathering and Soil Production. The time evolution of a periodic landscape under the influence of chemical weathering and physical erosion is computed. The model used incorporates weathering and soil production as a flux limited reaction controlled by groundwater flow. Scaling of the flow rate is obtained from a percolation theoretic treatment. The erosion of the soil material produced by this process is modeled by the diffusion of elevation, as consistent with downslope soil transport proportional to the tangent of the angle of the topography, and application of the equation of continuity to surface soil transport. Three initial topographies are examined over a periods of thousands of years and resulting landforms and soil productivity compared. -
Global Rates of Soil Production Independent of Soil Depth 2 Authors: Emma J
Non-peer reviewed preprint submitted to EarthArXiv 1 Global rates of soil production independent of soil depth 2 Authors: Emma J. Harrison1,2*, Jane K. Willenbring1,2, Gilles Y. Brocard3 3 Affiliations: 4 1 Scripps Institution of Oceanography, University of California San Diego, La Jolla, CA, USA 5 2 Now at Geological Sciences, Stanford University, Stanford, CA, USA 6 3Archéorient, UMR 5133, Maison de l’Orient et de la Mediterranée, University of Lyon 2, 7 France. 8 *corresponding author email : [email protected] 9 ABSTRACT 10 Accelerated rates of soil erosion threaten the stability of ecosystems1, nutrient cycles2, and 11 global food supplies3 if the processes that produce soil cannot keep pace. Over millennial 12 timescales, the rate of soil production is thought to keep pace with the rate of surface 13 erosion through negative feedbacks between soil thickness and the rate at which soil is 14 produced from the underlying mineral substrate4,5. This paradigm in the Earth Sciences 15 holds that some underlying mechanism lowers the rate of soil production when soil is thick 16 and increases the rate of soil production when soils are thin. This dynamic balance lends 17 support to two observations: First, soil covers >90% of Earth’s ice-free surface (NRCS) 18 despite global erosion rates that vary by three orders of magnitude3 and second, the 19 thickness of soils on Earth exists within a relatively narrow range even in old and deeply 20 weathered landscapes7. However, the actual coupling mechanism between soil thickness 21 and depth is unknown, and the functional form of the relationship is debated. -
Soil, Regolith, and Weathered Rock Theoretical Concepts and Evolution
Geoderma 368 (2020) 114261 Contents lists available at ScienceDirect Geoderma journal homepage: www.elsevier.com/locate/geoderma Soil, regolith, and weathered rock: Theoretical concepts and evolution in T old-growth temperate forests, Central Europe ⁎ Pavel Šamonila,b, , Jonathan Phillipsa,c, Pavel Daněka,d, Vojtěch Beneše, Lukasz Pawlika,f a Department of Forest Ecology, The Silva Tarouca Research Institute for Landscape and Ornamental Gardening, Lidická 25/27, 602 00 Brno, Czech Republic b Department of Forest Botany, Dendrology and Geobiocoenology, Faculty of Forestry and Wood Technology, Mendel University in Brno, Zemědělská 1, 613 00 Brno, Czech Republic c Earth Surface Systems Program, Department of Geography, University of Kentucky, Lexington, KY 40506, USA d Department of Botany and Zoology, Faculty of Science, Masaryk University, Kotlářská 267/2, 611 37 Brno, Czech Republic e G IMPULS Praha Ltd., Czech Republic f University of Silesia, Faculty of Earth Sciences, ul. Będzińska 60, 41-200 Sosnowiec, Poland ARTICLE INFO ABSTRACT Handling Editor: Alberto Agnelli Evolution of weathering profiles (WP) is critical for landscape evolution, soil formation, biogeochemical cycles, Keywords: and critical zone hydrology and ecology. Weathering profiles often include soil or solum (O, A, E, and Bhor- Soil evolution izons), non-soil regolith (including soil C horizons, saprolite), and weathered rock. Development of these is a Saprolite function of weathering at the bedrock weathering front to produce weathered rock; weathering at the boundary Weathering front between regolith and weathered rock to produce saprolite, and pedogenesis to convert non-soil regolith to soil. Hillslope processes Relative thicknesses of soil (Ts), non-soil regolith (Tr) and weathered rock (Tw) can provide insight into the Geophysical research relative rates of these processes at some sites with negligible surface removals or deposition. -
Rusell Review
European Journal of Soil Science, March 2019, 70, 216–235 doi: 10.1111/ejss.12790 Rusell Review Pedology and digital soil mapping (DSM) Yuxin Ma , Budiman Minasny , Brendan P. Malone & Alex B. Mcbratney Sydney Institute of Agriculture, School of Life and Environmental Sciences, The University of Sydney, Eveleigh, New South Wales, Australia Summary Pedology focuses on understanding soil genesis in the field and includes soil classification and mapping. Digital soil mapping (DSM) has evolved from traditional soil classification and mapping to the creation and population of spatial soil information systems by using field and laboratory observations coupled with environmental covariates. Pedological knowledge of soil distribution and processes can be useful for digital soil mapping. Conversely, digital soil mapping can bring new insights to pedogenesis, detailed information on vertical and lateral soil variation, and can generate research questions that were not considered in traditional pedology. This review highlights the relevance and synergy of pedology in soil spatial prediction through the expansion of pedological knowledge. We also discuss how DSM can support further advances in pedology through improved representation of spatial soil information. Some major findings of this review are as follows: (a) soil classes can bemapped accurately using DSM, (b) the occurrence and thickness of soil horizons, whole soil profiles and soil parent material can be predicted successfully with DSM techniques, (c) DSM can provide valuable information on pedogenic processes (e.g. addition, removal, transformation and translocation), (d) pedological knowledge can be incorporated into DSM, but DSM can also lead to the discovery of knowledge, and (e) there is the potential to use process-based soil–landscape evolution modelling in DSM. -
Using Constructed Soils for Green Infrastructure – Challenges and Limitations
SOIL, 6, 413–434, 2020 https://doi.org/10.5194/soil-6-413-2020 © Author(s) 2020. This work is distributed under SOIL the Creative Commons Attribution 4.0 License. Using constructed soils for green infrastructure – challenges and limitations Maha Deeb1,6,9, Peter M. Groffman1,3, Manuel Blouin2, Sara Perl Egendorf1,3, Alan Vergnes4, Viacheslav Vasenev7,6, Donna L. Cao3, Daniel Walsh5, Tatiana Morin6, and Geoffroy Séré8 1Advanced Science Research Center, Graduate Center, City University of New York, 10031 New York, NY, USA 2Agroécologie, AgroSup Dijon, INRA, University of Bourgogne Franche-Comté, 21078 Dijon, France 3Department of Earth and Environmental Sciences, Brooklyn College, City University of New York, 11210 Brooklyn, NY, USA 4Department of Biology, Ecology and Environment, Université Paul-Valéry (Montpellier 3), 34090 Montpellier, France 5Lamont-Doherty Earth Observatory, Columbia University, 10964 Palisades, NY, USA 6New York City Urban Soils Institute, Brooklyn College, City University of New York, 11210 Brooklyn, NY, USA 7Department of Landscape Design and Sustainable Ecosystems, Peoples’ Friendship University of Russia (RUDN), 117198 Moscow, Russia 8Laboratoire Sols et Environnement, Université de Lorraine, INRA, UMR 1120, 54518 Vandœuvre-lès-Nancy, France 9Laboratoire Interdisciplinaire des Environnements Continentaux, Université de Lorraine, UMR 7360 CNRS, 57070 Metz, France Correspondence: Maha Deeb ([email protected]) Received: 10 November 2019 – Discussion started: 13 December 2019 Revised: 14 July 2020 – Accepted: 29 July 2020 – Published: 8 September 2020 Abstract. With the rise in urban population comes a demand for solutions to offset environmental problems caused by urbanization. Green infrastructure (GI) refers to engineered features that provide multiecological func- tions in urban spaces. -
Erosion and Non-Steady State Storage of Soil Organic Carbon on Undisturbed Hillslopes in Coastal California
Erosion and Non-steady State Storage of Soil Organic Carbon on Undisturbed Hillslopes in Coastal California Kyungsoo Yooa1, Ronald Amundsona, Arjun M. Heimsathb, William E. Dietrichc aDivision of Ecosystem Sciences, University of California at Berkeley, CA 94720, USA bDepartment of Earth Sciences, Dartmouth College, Hanover, NH 08755, USA cDepartment of Earth and Planetary Sciences, University of California at Berkeley, CA 94720, USA [email protected] Introduction Sites and Measurements Result 2: C Accumulation in Depositional Slope Most upland ecosystems under natural vegetation are not level; land with < 8% slope On the following hillslopes at two sites, we made detailed measurements of the spatial variation in soil organic carbon content and above ground net primary productivity Fig.10 Soil Accumulation in the Tennessee Valley Hollow comprises only 36% of global soil [Staub and Rosenzweig, 1992]. These hilly upland (ANPP). The soil %C was combined with the erosion mechanism on the site to determine the %C of eroding soils. We also made detailed topographic survey with total Eroded soils accumulated in depositional slopes that were estimated to have residence times of (Eq.3) landscapes represent unexplored processes relevant to the global C cycle. In recent laser station. The resulting data was used to calculate the local slope curvature over the entire hillslopes. 13 kyrs at TV and 5.3 kyrs at BD. In the TV hollow (depositional slope within a zero order 200 years, geomorphic research on the processes of soil transport on soil mantled Current Thickness Table 1 watershed), 15-24 kg C m-2 of soil C has accumulated at a long-term rate of hillslopes has provided an empirically constrained mathematical framework to explore Tennessee Valley Black Diamond Fig.3 -2 -1 Tennessee Valley Fig.4 Mediterranean Mediterranean 1.6-1.9 g C m yr . -
Soils As Pacemakers and Limiters of Global Silicate Weathering
Originally published as: Dixon, J. L., von Blanckenburg, F. (2012): Soils as pacemakers and limiters of global silicate weathering. ‐ Comptes Rendus Geoscience, 344, 11 ‐ 12, 597‐609 DOI: 10.1016/j.crte.2012.10.012 Soils as pacemakers and limiters of global silicate weathering Jean L. Dixon1,2 and Friedhelm von Blanckenburg1 1 Helmholtz Centre Potsdam, GFZ German Centre for Geosciences, Telegraphenberg, 14473 Potsdam, Germany 2 Department of Geography, University of California, Santa Barbara, CA 93106, USA Keywords: chemical weathering, soil production, speed limits, erosion, regolith, river fluxes Comptes rendus – Geosciences 344, 597-609, 2012 Abstract The weathering and erosion processes that produce and destroy regolith are widely recognized to be positively correlated across diverse landscapes. However, conceptual and numerical models predict some limits to this relationship that remain largely untested. Using new global data compilations of soil production and weathering rates from cosmogenic nuclides and silicate weathering fluxes from global rivers, we show that the weathering- erosion relationship is capped by certain ‘speed limits’. We estimate a soil production speed limit of between 320 to 450 t km-2 y-1 and the associated weathering rate speed limit of roughly 150 t km-2 y-1. These limits appear to be valid for a range of lithologies, and also extend to mountain belts, where soil cover is not continuous and erosion rates outpace soil production. We argue that the presence of soil and regolith is a requirement for high weathering fluxes from a landscape, and that rapidly eroding, active mountain belts are not the most efficient sites for weathering. -
445 10Be, 14C Distribution, and Soil Production Rate in A
RADIOCARBON, Vol 46, Nr 1, 2004, p 445–454 © 2004 by the Arizona Board of Regents on behalf of the University of Arizona 10BE, 14C DISTRIBUTION, AND SOIL PRODUCTION RATE IN A SOIL PROFILE OF A GRASSLAND SLOPE AT HESHAN HILLY LAND, GUANGDONG CD Shen1,2 • J Beer3 • S Ivy-Ochs4 • Y Sun1 • W Yi1 • P W Kubik5 • M Suter4 • Z Li6 • S Peng5 • Y Yang1 ABSTRACT. Concentrations of organic carbon, carbon isotopes (13C and 14C), atmospheric 10Be in soil, and in situ 10Be in bedrock and weathering rock were determined in a study of a profile of a grassland slope at the Heshan Hilly Land Interdis- ciplinary Experimental Station, Chinese Academy of Sciences, in Guangdong Province, China. A good linear relationship between depth and the 14C apparent age of the organic carbon demonstrates that the rock weathering process and the accu- mulation process of organic matter in the slope are relatively stable. Both 14C and 10Be results show that about 34% of soil in the grassland slope has been eroded during the past 3800 yr. The 10Be results for interstitial soil from weathered rocks show that the 90-cm-thick weathering rock layer above the bedrock has evolved over a period of 1.36 Myr. The concentrations of in situ 10Be in the weathered rock and bedrock are 10.7 × 104 atoms/g and 8.31 × 104 atoms/g, respectively. The weathering rate of the bedrock, equivalent to the soil production rate, was estimated at 8.8 × 10–4 cm/yr, and the exposure ages of the weathered rock and the bedrock were 72 kyr and 230 kyr, respectively. -
The Effect of Layered Lithology on Soil Formation and Landscape Evolution a Case Study in the Flint Hills, Kansas, USA
The effect of layered lithology on soil formation and landscape evolution A case study in the Flint Hills, Kansas, USA Marte Stoorvogel March, 2019 The effect of layered lithology on soil formation and landscape evolution A case study in the Flint Hills, Kansas, USA M.M. (Marte) Stoorvogel 951113808060 Master thesis Earth and Environment: Soil Geography and Earth Surface Dynamics March, 2019 Wageningen, The Netherlands Soil Geography and Landscape group Wageningen UR Supervisors: W.M. (Marijn) van der Meij MSc Soil Geography and Landscape group Wageningen UR, Netherlands Dr. A.J.A.M (Arnaud) Temme Department of Geography Kansas State University, USA ii Abstract Soil-landscape relations originate from interactions between pedogenic and geomorphic processes. These relations can especially be strong in systems with a layered bedrock lithology as a result of the strong influence of parent material on soil formation. However, previous research has not yet focused on feedbacks between soil and landscape evolution in layered bedrock systems. Therefore, the objective of this research was to get a better understanding of the influence of lithology on soil formation and landscape evolution across hillslopes on layered bedrock. This study was conducted at the Konza Prairie ecological research station in Kansas, USA, where layers of limestone and shale alternate. A field survey, laboratory analysis and model study were performed to reach the objective. Results show that soil properties vary strongly across hillslopes on layered bedrock and that differences in elevation, slope steepness, profile curvature and parent material affect these properties. Especially soil depth, surface stoniness and vegetation coverage follow a clear pattern across the hillslopes, with thin soils, high surface stoniness and low vegetation coverage at convex positions and steep slopes.