Jopcs632(2015)012034.Pdf
Total Page:16
File Type:pdf, Size:1020Kb
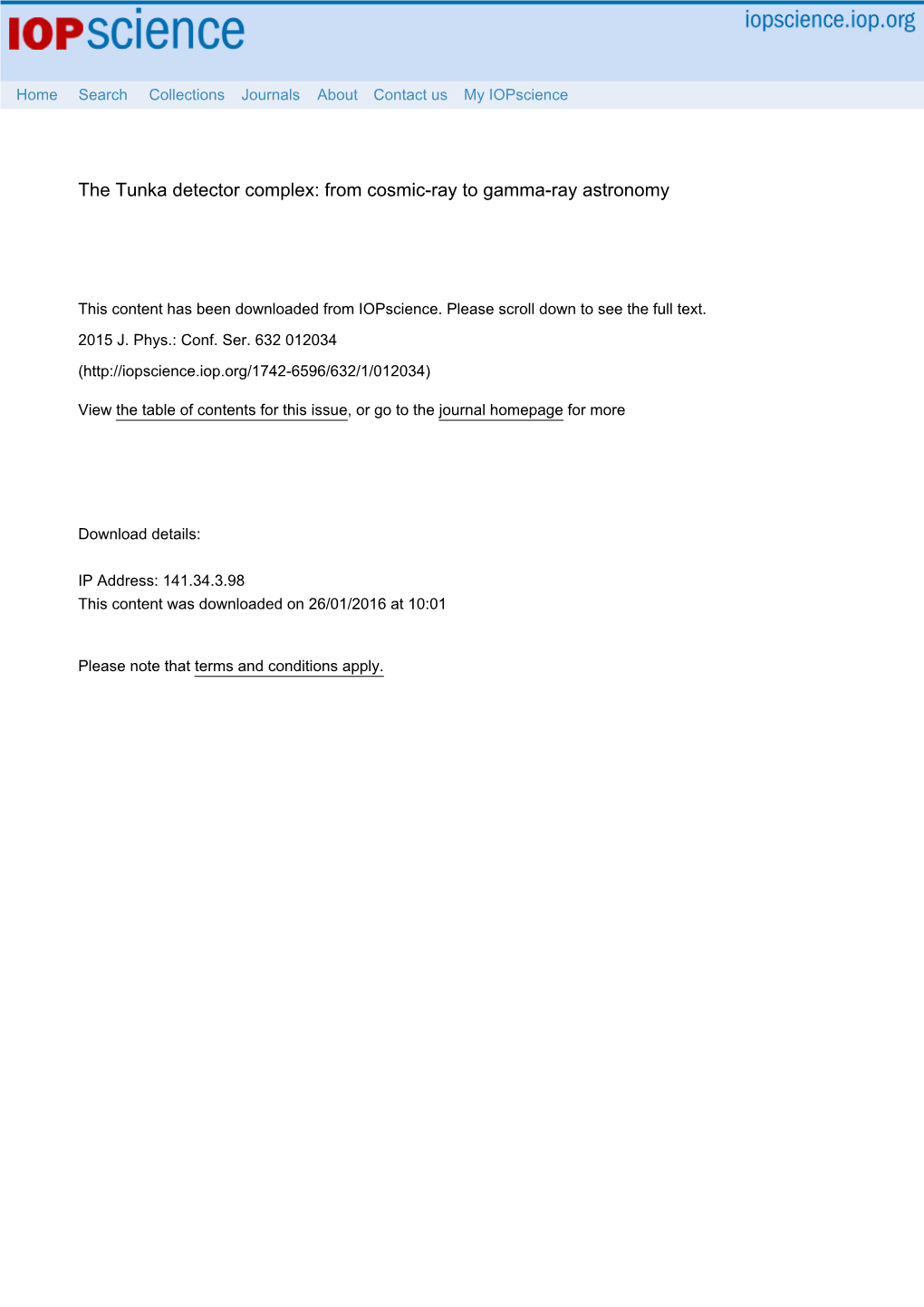
Load more
Recommended publications
-
Cherenkov Light Imaging in Astroparticle Physics
Cherenkov light imaging in astroparticle physics U. F. Katz Erlangen Centre for Astroparticle Physics, Friedrich-Alexander University Erlangen-N¨urnberg, Erwin-Rommel-Str. 1, 91058 Erlangen, Germany Abstract Cherenkov light induced by fast charged particles in transparent dielectric media such as air or water is exploited by a variety of experimental techniques to detect and measure extraterrestrial particles impinging on Earth. A selection of detection principles is discussed and corresponding experiments are presented together with breakthrough-results they achieved. Some future develop- ments are highlighted. Keywords: Astroparticle physics, Cherenkov detectors, neutrino telescopes, gamma-ray telescopes, cosmic-ray detectors 1. Introduction Photomultiplier tubes (PMTs) and, more recently, silicon pho- tomultipliers (SiPMs) [3,4] are the standard sensor types. They In 2018, we commemorate the 60th anniversary of the award are provided by specialised companies who cooperate with the of the Nobel Prize to Pavel Alexeyewich Cherenkov, Ilya experiments in developing and optimising sensors according to Mikhailovich Frank and Igor Yevgenyevich Tamm for the dis- the respective specific needs (see e.g. [5,6]). covery and the interpretation of the Cherenkov effect [1,2]. In the following, the detection principles of different types of The impact of this discovery on astroparticle physics is enor- Cherenkov experiments in astroparticle physics are presented mous and persistent. Cherenkov detection techniques were ins- together with selected technical details and outstanding results. tumental for the dicovery of neutrino oscillations; the detection of high-energy cosmic neutrinos; the establishment of ground- based gamma-ray astronomy; and important for the progress in 2. Ground-based gamma-ray detectors cosmic-ray physics. -
From Cosmic Ray to Gamma-Ray Astronomy
The Tunka experiment: from cosmic ray to gamma-ray astronomy. N.Budnev, Irkutsk State University For Tunka&TAIGA - collaboration High energy charged particles and gamma – ray detections - Charged particles - Cherenkov light P, N, For electrons with - Fluorescence light γ -Radio emission Ee >25 MeV Ve > C/ n Atmosphere as a huge calorimeter EAS Energy EAS Cherenkov light g wide-angle detection E = A · [Nph (200m)] Density of Cherenkov light at distance 200 m from technique θ, φ core g = 0.94±0.01 (for 10 16 – 10 18 eV) Xmax Average CR Xmax = C –D· lg τ (400) mass A (τ(400) - width of a Cherenkov pulse LnA ~ Xmax at distance 400 m EAS core from) Xmax = F( P) X0 P -Steepness of a Lateral Distribution Function (LDF ) Tunka-133 array: 175 optical detectors distributed on 3 km2 area 1 км 50 km from Lake Baikal Tunka Collaboration S.F.Beregnev, S.N.Epimakhov, N.N. Kalmykov, N.I.KarpovE.E. Korosteleva, V.A. Kozhin, L.A. Kuzmichev , M.I. Panasyuk, E.G.Popova, V.V. Prosin, A.A. Silaev, A.A. Silaev(ju), A.V. Skurikhin, L.G.Sveshnikova I.V. Yashin, Skobeltsyn Institute of Nucl. Phys. of Moscow State University, Moscow, Russia ; N.M. Budnev, O.A. Chvalaev, O.A. Gress, A.V.Dyachok, E.N.Konstantinov, A.V.Korobchebko, R.R. Mirgazov, L.V. Pan’kov, A.L.Pahorukov, Yu.A. Semeney, A.V. Zagorodnikov Institute of Applied Phys. of Irkutsk State University, Irkutsk, Russia ; B.K. Lubsandorzhiev, B.A. Shaibonov(ju) , N.B. Lubsandorzhiev Institute for Nucl. -
Radio Measurements of the Energy and the Depth of the Shower Maximum of Cosmic-Ray Air Showers by Tunka-Rex
Home Search Collections Journals About Contact us My IOPscience Radio measurements of the energy and the depth of the shower maximum of cosmic-ray air showers by Tunka-Rex This content has been downloaded from IOPscience. Please scroll down to see the full text. JCAP01(2016)052 (http://iopscience.iop.org/1475-7516/2016/01/052) View the table of contents for this issue, or go to the journal homepage for more Download details: IP Address: 131.169.95.182 This content was downloaded on 09/01/2017 at 10:16 Please note that terms and conditions apply. You may also be interested in: The wavefront of the radio signal emitted by cosmic ray air showers W.D. Apel, J.C. Arteaga-Velázquez, L. Bähren et al. Tunka-Rex: a Radio Extension of the Tunka Experiment F G Schröder, D Besson, N M Budnev et al. Radio Measurements of Air Showers with LOPES F G Schröder, W D Apel, J C Arteaga-Velazquez et al. High-energy cosmic rays and the Greisen–Zatsepin–Kuz'min effect A A Watson Reconstruction of inclined air showers detected with thePierre Auger Observatory The Pierre Auger collaboration Polarized radio emission from extensive air showers measured with LOFAR P. Schellart, S. Buitink, A. Corstanje et al. Search for the end of the energy spectrum of primary cosmic rays M Nagano Cosmic-Ray Energy Spectrum1017-1018.3 eV T. Abu-Zayyad, K. Belov, D. J. Bird et al. ournal of Cosmology and Astroparticle Physics JAn IOP and SISSA journal Radio measurements of the energy and the depth of the shower maximum of cosmic-ray air showers by JCAP01(2016)052 Tunka-Rex The Tunka-Rex collaboration P.A. -
From Cosmic Ray to Gamma-Ray Astronomy in the Tunka Valley
Home Search Collections Journals About Contact us My IOPscience The TAIGA experiment: from cosmic ray to gamma-ray astronomy in the Tunka valley This content has been downloaded from IOPscience. Please scroll down to see the full text. 2016 J. Phys.: Conf. Ser. 718 052006 (http://iopscience.iop.org/1742-6596/718/5/052006) View the table of contents for this issue, or go to the journal homepage for more Download details: IP Address: 129.13.72.197 This content was downloaded on 09/08/2017 at 07:34 Please note that terms and conditions apply. You may also be interested in: The Tunka Radio Extension: Latest Analysis Results D Kostunin, P A Bezyazeekov, N M Budnev et al. The Tunka detector complex: from cosmic-ray to gamma-ray astronomy N Budnev, I Astapov, N Barbashina et al. The Tunka-Grande experiment R.D. Monkhoev, N.M. Budnev, A. Chiavassa et al. TAIGA the Tunka Advanced Instrument for cosmic ray physics and Gamma Astronomy — present status and perspectives. N M Budnev, I I Astapov, A G Bogdanov et al. The HiSCORE experiment and its potential for gamma-ray astronomy M Tluczykont, D Hampf, U Einhaus et al. Tunka-Rex: a Radio Extension of the Tunka Experiment F G Schröder, D Besson, N M Budnev et al. THE RESULTS OF PHOTOMETRIC RECORDING OF THE OCCULTATION OF THE STAR HIP 97157 BY ASTEROIDDAPHNE WITH (41) THE TELESCOPE OF THE GLOBAL MASTER ROBOTIC NET E. M. Trunkovsky, E. S. Gorbovskoy, D. V. Denisenko et al. First deployment and prototype data of HiSCORE R Nachtigall, M Kunnas, S N Epimakhov et al. -
Exploring Cosmic Ray Origins with Ground-Based EAS Arrays Tunka and Hiscore
Exploring cosmic ray origins with ground-based EAS arrays Tunka and HiSCORE Sergey Epimakhov Exploring cosmic ray origins with ground-based EAS arrays Tunka and HiSCORE Dissertation with the aim of achieving a doctoral degree at the Faculty of Mathematics, Informatics and Natural Sciences Department of Physics University of Hamburg Submitted by Sergey Epimakhov Hamburg 2015 Gutachter der Dissertation: Prof. Dr. Dieter Horns Dr. Vasily Prosin Gutachter der Disputation: Prof. Dr. G¨unter Sigl Prof. Dr. Caren Hagner Dr. Martin Tluczykont Datum der Disputation: 24.09.15 Dekan der MIN Fakult¨at: Prof. Dr. Heinrich Graener Dedicated to my family Per aspera ad astra Abstract The main properties of cosmic radiation over the last hundred years have been studied with good accuracy and are not in doubt: cosmic rays are nuclei of almost all elements of the periodic table with a small fraction of other particles coming from the outer space at Earth with the non-thermal energy spectrum from 106 to 1020 eV. However, the source of cosmic rays still remains unknown. To examine the question of the origin of cosmic rays neutral particles are perfectly suited as messengers. Thereby the gamma ray sky in the range above 10 { 100 TeV is of great interest. Hypothetical galactic accelerators, pevatrons, must have gamma ray spectra up to these energies. A new non-imaging wide-angle Cherenkov experiment HiSCORE is aimed at search- ing the ultra-high energy gamma rays above 30 TeV and measuring the primary spectrum and mass composition of cosmic rays above 100 TeV with a unprece- dented accuracy. -
Status and Perspectives of Astroparticle Physics in Europe
SStatustatus aandnd PPerspectiveerspective ooff AAstroparticlestroparticle PPhysicshysics inin EEuropeurope Astroparticle Physics Roadmap Phase I ASPERA Roadmap • Phase I • Astroparticle physics for Europe 2 ASPERA Roadmap • Phase I • On this document The Steering Committee of ApPEC (Astroparticle Physics European Coordination) has charged the ApPEC Peer Review Committee (PRC) in 2005 to prepare a roadmap for astroparticle physics in Europe* which covers the next decade. The need for such a roadmap arises since projects in astroparticle physics move to ever larger sensitivity and scale, with costs of individual projects on the 100 M€ scale or beyond. The roadmap document presented here was prepared by the PRC between October 2005 and January 2007. As a first step towards the roadmap, the state of the experiments in the field was evaluated using a questionnaire filled out by the spokespersons of all astroparticle experiments in Europe, or with European participation (see Appendix 2). Based on this information, on input from the proponents and on presentations given to ApPEC in the last years, the most promising research areas and instrumental approaches were identified. A town meeting in Munich in 2005 served to discuss and iterate these initial concepts with the community at large. The recommendations given in the roadmap were in several stages iterated in particular with the spokespersons of the experiments as well as with the ApPEC Steering Committee. After a large meeting in Valencia (Nov.7/8, 2006), further significant modifications have been prepared by smaller working groups, submitted by individuals and included in the present version. The resulting ApPEC roadmap marks the first stage (ASPERA Roadmap/Stage I) of a strategy process which foresees a “final” roadmap (ASPERA Roadmap/Stage 3) in July 2008. -
Cherenkov Experiments in the Tunka Valley
The Tunka Experiment: Cosmic Ray and Multi-TeV Gamma-Ray Astronomy Arrays in the Tunka Valley Bayarto Lubsandorzhiev Institute for Nuclear Research of RAS, Moscow Russia for TUNKA and TAIGA Collaborations Outline Introduction Tunka-25 Tunka-133 TAIGA (Tunka Advanced Internatinal Gamma and cosmic ray Array) Victor Hess 1912 г. – the discovery of cosmic rays On a balloon at an altitude of 5000 meters Victor Hess discovered “penetrating radiation” coming from space. Nobel prize 1936 The origin of cosmic rays? – XX-XXI century mystery! Where from? - Energy? Where? - Sources? How? - Acceleration mechanisms? Detection of Cherenkov light from EAS Cherenkov experiments in the Tunka Valley Tunka Valley “…..till Baikal it is the Siberia’s dull prose, just from Baikal the Siberian delightful poetry starts….” A.P.Chekhov (Letters from Siberia) Cherenkov experiments in the Tunka Valley 1993 - ………. 1991-1992: first experiments at the Lake Baikal ice with QUASAR-370 photodetectors (Bezrukov, Kuzmichev, Lubsandorzhiev et al.) 1993г. - Move to the Tunka Valley. (Bezrukov, Budnev, Kuzmichev, Lubsandorzhiev, Pokhil et al.) SMECA: Surface Mobile Eas Cherenkov Array Kuzmichev, Lubsandorzhiev, Pokhil et al Eth~400 TeV, <>~0.5 Tunka Valley, Buryatia Republic Tunka-4 4 QUASAR-370 phototubes Bezrukov, Kuzmichev, Lubsandorzhiev, Pokhil et al. 24th ICRC 1995 Rome. “Knee” in the energy spectrum at 31015 эВ. TUNKA-25 COLLABORATION E.Korosteleva, L.Kuzmichev, V.Prosin, I.Yashin Scobeltsyn Institute of Nuclear Physics of MSU (Moscow, Russia) N.Budnev, O.Gress, L.Pankov, -
Acoustic and Radio Eev Neutrino Detection Activities Book of Abstracts
ARENA 2012 - Acoustic and Radio EeV Neutrino Detection Activities Tuesday 19 June 2012 - Friday 22 June 2012 Erlangen Castle (centre of town) Book of abstracts ARENA 2012 - Acoustic and Radio EeV Neutrino Detection Activities / Book of abstracts Monday 18 June 2012 Table of contents Introduction ............................................................................................................................... 1 Welcome Address ....................................................................................................................... 1 Radio Detection in Dense Matter: Balloon, telescope, satellite ............................................................... 1 LUNASKA neutrino search with the Parkes and ATCA telescopes ......................................................... 1 Searching for neutrino radio flashes from the Moon with LOFAR ......................................................... 2 Lunar Imaging and Ionospheric Calibration for the Lunar Cherenkov Technique ..................................... 2 Lunar Space Missions for Observation of Ultrahigh-Energy Cosmic Rays and Neutrinos ............................ 3 Overview of MHz air shower radio experiments and results ................................................................. 3 Recent developments at the Auger Engineering Radio Array ................................................................ 3 Energy Estimation for Cosmic Rays Measured with the Auger Engineering Radio Array ........................... 4 Spectral index analysis of the data -
Very-High Energy Gamma-Ray Astronomy
Very-high energy gamma-ray astronomy A 23-year success story in high-energy astroparticle physics Eckart Lorenz1;a and Robert Wagner1;2;b 1 Max-Planck-Institut fur¨ Physik, Fohringer¨ Ring 6, 80805 Munchen,¨ Germany 2 Excellence Cluster “Origin and Structure of the Universe”, Boltzmannstraße 2, 85748 Garching b. Munchen,¨ Ger- many Abstract. Very-high energy (VHE) gamma quanta contribute only a minuscule fraction – be- low one per million – to the flux of cosmic rays. Nevertheless, being neutral particles they are currently the best “messengers” of processes from the relativistic/ultra-relativistic Universe because they can be extrapolated back to their origin. The window of VHE gamma rays was opened only in 1989 by the Whipple collaboration, reporting the observation of TeV gamma rays from the Crab nebula. After a slow start, this new field of research is now rapidly expanding with the discovery of more than 150 VHE gamma-ray emitting sources. Progress is intimately related with the steady improvement of detectors and rapidly increasing computing power. We give an overview of the early attempts before and around 1989 and the progress after the pio- neering work of the Whipple collaboration. The main focus of this article is on the development of experimental techniques for Earth-bound gamma-ray detectors; consequently, more empha- sis is given to those experiments that made an initial breakthrough rather than to the successors which often had and have a similar (sometimes even higher) scientific output as the pioneering experiments. The considered energy threshold is about 30 GeV. At lower energies, observa- tions can presently only be performed with balloon or satellite-borne detectors. -
Study for the Wide-Angle Air Cherenkov Detector Hiscore and Time Gradient Event Reconstruction for the H.E.S.S
Study for the wide-angle air Cherenkov detector HiSCORE and time gradient event reconstruction for the H.E.S.S. experiment Dissertation zur Erlangung des Doktorgrades des Department Physik der Universitat¨ Hamburg vorgelegt von Daniel Hampf aus Recife (Brasilien) Hamburg 2012 Gutachter der Dissertation: Prof. Dr. Dieter Horns Prof. Dr. Bruce Dawson Gutachter der Disputation: Prof. Dr. Erika Garutti Dr. Axel Lindner Datum der Disputation: 22.05.2012 Vorsitzender des Prufungsausschusses:¨ Dr. Georg Steinbruck¨ Vorsitzender des Promotionsausschusses: Prof. Dr. Peter Hauschildt Dekan der MIN Fakultat:¨ Prof. Dr. Heinrich Graener If I can see any further, it is only because I am standing on the shoulders of giants. after Bernard of Chartres Dedicated to the memory of the countless men and women who throughout the centuries have devoted their lives to the quest for truth, the advancement of reason and the fight against ignorance. Only the pursuit of enlightenment has enabled humankind to rise above the received conventions of existence, into the boundless space of intellect. Abstract This thesis presents efforts to extend the range of astronomical gamma-ray observations to the ultra high en- ergy regime, i.e. to energies above 3×1013 eV. Current gamma-ray instruments like the H.E.S.S. Cherenkov telescope array have limited sensitivity towards those energies, since the flux of gamma-ray sources is ex- tremely small in this regime and not enough events can be detected with the available effective areas. It is shown that the potential of H.E.S.S. for the observation at ultra high energies can be improved by utilising the data on the time evolution of the recorded Cherenkov light images. -
TAIGA - an Advanced Hybrid Detector Complex for Astroparticle Physics and High Energy Gamma-Ray Astronomy in the Tunka Valley
TAIGA - an advanced hybrid detector complex for astroparticle physics and high energy gamma-ray astronomy in the Tunka valley. N.Budnev, Irkutsk State University For TAIGA collaboration At present an Imaging Atmospheric Cherenkov Telescopes (IACT) are the main instruments for the ground based high energy gamma astronomy An Imaging Atmospheric camera Mirror with Cherenkov Telescope diameter 4-24 m (IACT) - narrow-angle telescope (3-5 FOV) with a mirror of 4 -24 m diameter which reflects EAS Cherenkov light into a camera with up to 1000 PMT where Cherenkov EAS image is formed. H.E.S.S. camera (D = 3 m!) EAScamera Imaging Atmospheric Cherenkov Arrays (2-5 IACT) Whipple HEGRA H.E.S.S. MAGIC VERITAS S ~ 0.1 km2 About 200 sources of gamma rays with energy more than 1 TeV were discovered with IACT arrays. But a few gamma quantum with energy more then 50 TeV were detected up to now. An area of an array should be a few square kilometers as minimum to detect high energy gamma rays. EAS Cherenkov light detection technique by wide angle arrays in the Tunka - Experiment EAS Energy Average CR mass A θ, φ g LnA ~ Xmax E = A · [Nph(200m)] Xmax g = 0.94±0.01 Xmax = C –D· lg τ (400) (τ(400) - width of a Cherenkov pulse at distance 400 m EAS core from) Xmax = F(P) X0 P -Steepness of a Lateral Distribution Function (LDF) Tunka-133 array: 175 Cherenkov detectors distributed on 3 km2 area, in operation since 2009y 1 км 50 km from Lake Baikal Advantage of the Tunka-133 array: 1. -
Design of the LED Driver for the Precision Optical Calibration Module
Design of the LED Driver for the Precision Optical CAlibration Module Master Thesis Antonio Becerra Esteban Technische Universit¨atM¨unchen Physics Department Chair for Experimental Physics with Cosmic Particles December 4, 2017 Supervisor: Prof. Dr. Elisa Resconi Abstract A study of the circuit designed by J.S.Kapustinsky et Al. in 1985 as a cheap fast flasher for the Precision Optical CAlibration Module (POCAM) is carried out. Its characteristics make it a perfect candidate as short light pulses generator in order to simulate neutrino double-bang-like events in the framework of the next IceCube upgrade, IceCube-Gen2. The response of diverse LEDs with different features was studied by varying the available charge, the bias voltage and the inductance of the Kapustinsky circuit. The pulses were measured with a PIN-Diode and a SiPM. The amplification of the signal was accomplished by a couple of charge sensitive preamplifiers and afterwards monitored and stored in the oscilloscope for further analysis. To produce an isotropic pulse, the LED flashed inside a PFTE sphere that spread the light in 4π. All the tests were carried out inside a dark metallic box with foam cladding to ensure the isolation from external photon sources. For a first test at the Gigaton Volume Detector (GVD) at Lake Baikal four different Kapustinky-like circuits were set up. This configuration allows the POCAM to produce four different light pulses: two long pulses (15 − 25 ns) and two short pulses (5 − 10 ns) in green (525 − 527 nm) and blue (455 − 470 nm). The final driver board includes the PIN-Diode and the SiPM as well as the preamplifiers that sends the information to an FPGA for its storage.