BRCA1 Localization to the Telomere and Its Loss from the Telomere In
Total Page:16
File Type:pdf, Size:1020Kb
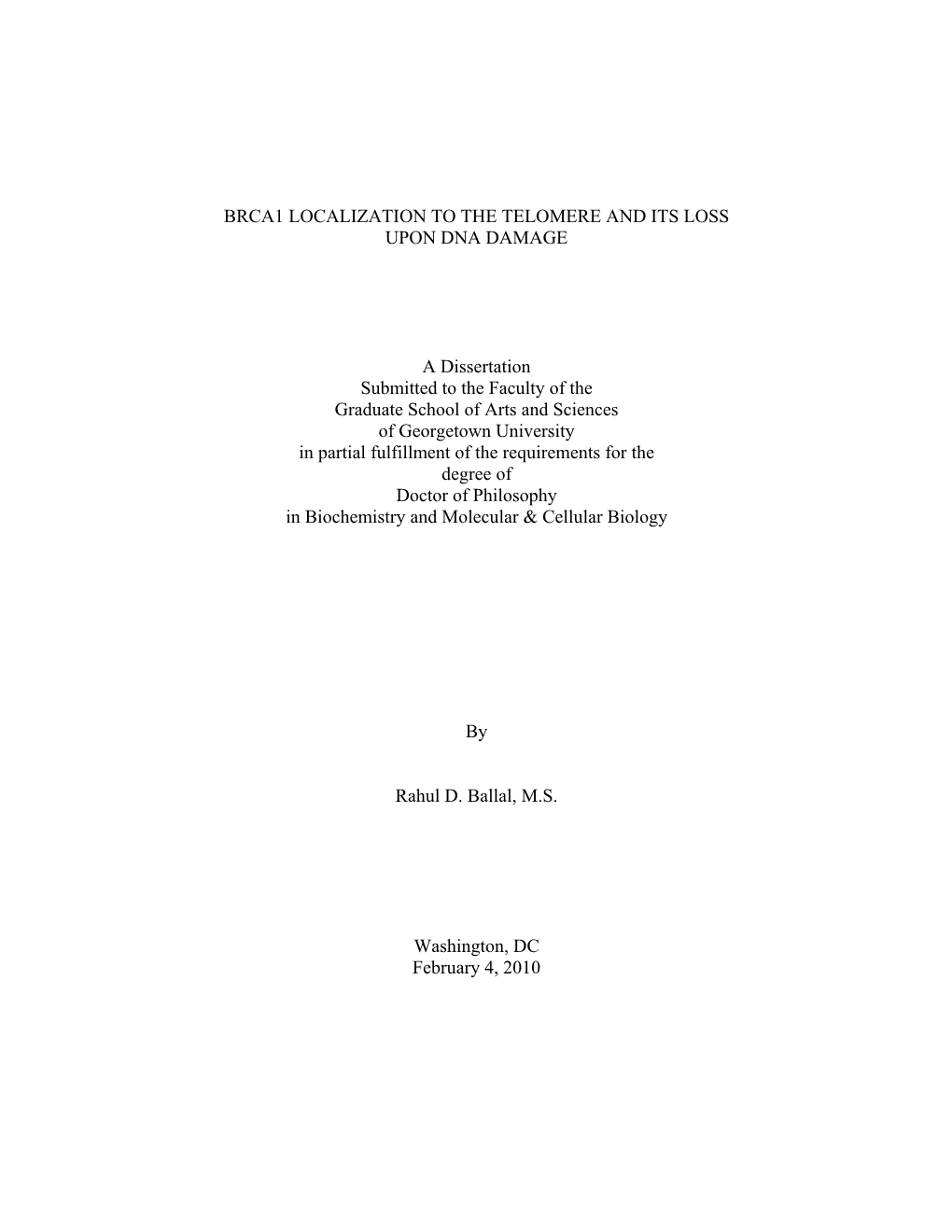
Load more
Recommended publications
-
BRCA1 in Hormonal Carcinogenesis: Basic and Clinical Research
Endocrine-Related Cancer (2005) 12 533–548 REVIEW BRCA1 in hormonal carcinogenesis: basic and clinical research E M Rosen, S Fan and C Isaacs Department of Oncology, Lombardi Cancer Center, Georgetown University, 3970 Reservoir Road, NW, Washington, District of Columbia 20057, USA (Requests for offprints should be addressed to E M Rosen; Email: [email protected]) Abstract The breast and ovarian cancer susceptibility gene-1 (BRCA1) located on chromosome 17q21 encodes a tumor suppressor gene that functions, in part, as a caretaker gene in preserving chromosomal stability. The observation that most BRCA1 mutant breast cancers are hormone receptor negative has led some to question whether hormonal factors contribute to the etiology of BRCA1-mutant breast cancers. Nevertheless, the caretaker function of BRCA1 is a generic one and does not explain why BRCA1 mutations confer a specific risk for tumor types that are hormone- responsive or that hormonal factors contribute to the etiology, including those of the breast, uterus, cervix, and prostate. An accumulating body of research indicates that in addition to its well- established roles in regulation of the DNA damage response, the BRCA1 protein interacts with steroid hormone receptors (estrogen receptor (ER-a) and androgen receptor (AR)) and regulates their activity, inhibiting ER-a activity and stimulating AR activity. The ability of BRCA1 to regulate steroid hormone action is consistent with clinical-epidemiological research suggesting that: (i) hormonal factors contribute to breast cancer risk in BRCA1 mutation carriers; and (ii) the spectrum of risk-modifying effects of hormonal factors in BRCA1 carriers is not identical to that observed in the general population. -
Functional Analysis of Novel Β-Catenin Mutants
AN ABSTRACT OF THE THESIS OF Mohamed B. Al-Fageeh for the degree of Master of Science in Biochemistryand Biophysics presented on February 12, 2003. Title: Functional Analysis of Novel -catenin Mutants Abstract approved Redacted for privacy Roderick H. Dashwood 13-catenin is a multi functional protein that is involved in cell-cell adhesion and cell signaling. In non-stimulated cells,-catenin is tightly down-regulated by GSK-3dependent phosphorylation at Ser and Thr residues, followed by rapid ubiquitination and proteasomal degradation. It is well established that mutations within the regulatory GSK-313 region lead to stabilized13-catenin and constitutive f3-cateninlTCF-dependent gene activation. Furthermore, it has been shown that amino acids adjacent to codon 33, namely 32 and 34 of -catenin,are hotspots for substitution mutations in carcinogen-induced animaltumors. Thus, a major hypothesis of this thesis was that substitution mutations at codon 32 of13-catenin interfere with phosphorylation and ubiquitination of -catenin. Site-directed mutagenesis was used to create defined-catenin mutants, namely D32G, D32N, and D32Y. The signaling potential of various13-catenin was analyzed in a gene reporter assay by co-transfection witha hTcf eDNA with a reporter plasmid containing a Tcf-dependent promoter (TOPFlash). Therewas a significant enhancement of the reportergene activity with all [3-catenin mutants compared to WT t3-catenin after 48 hours of transfection. Protein analysisby Western blotting showed massive accumulation of mutant-catenin. Antibody specific for phosphorylated 13-catenin showed that the accumulated D32G and D32N 13-catenin proteins were strongly phosphorylated bothin vivoandin vitro, whereas D32Y 13-catenin exhibited significantly attenuated phosphorylationin vivo. -
Original Article Gastrointestinal Stem Cells and Cancer
Stem Cell Reviews Copyright © 2005 Humana Press Inc. All rights of any nature whatsoever are reserved. ISSN 15501550-8943/05/1:1–10/$30.00‐8943 / 05 / 1:233‐241 / $30.00 Original Article Gastrointestinal Stem Cells and Cancer— Bridging the Molecular Gap S.J. Leedham,*,1 A.T.Thliveris,2 R.B. Halberg,2 M.A. Newton,3 and N.A.Wright1 1Histopathology Unit, Cancer Research UK, 44 Lincoln’s Inn Fields, London WC2A 3PX,UK; 2McArdle Laboratory for Cancer Research, University of Wisconsin—Madison,Madison,WI 53706; and 3Department of Statistics, University of Wisconsin—Madison, Madison WI 53706 Abstract Cancer is believed to be a disease involving stem cells. The digestive tract has a very high cancer prevalence partly owing to rapid epithelial cell turnover and exposure to dietary toxins. Work on the hereditary cancer syndromes including famalial adenoma- tous polyposis (FAP) has led to significant advances, including the adenoma-carcinoma sequence. The initial mutation involved in this stepwise progression is in the “gate- keeper” tumor suppressor gene adenomatous polyposis coli (APC). In FAP somatic, second hits in this gene are nonrandom events, selected for by the position of the germ- line mutation. Extensive work in both the mouse and human has shown that crypts are clonal units and mutated stem cells may develop a selective advantage, eventually form- ing a clonal crypt population by a process called “niche succession.” Aberrant crypt foci are then formed by the longitudinal division of crypts into two daughter units—crypt fission. The early growth of adenomas is contentious with two main theories, the “top- down” and “bottom-up” hypotheses, attempting to explain the spread of dysplastic tissue in the bowel. -
Stochastic Modelling of Tumorigenesis in P53 Deficient Mice
British Joumal of Cancer (1998) 77(2), 243-252 © 1998 Cancer Research Campaign Stochastic modelling of tumorigenesis in p53 deficient mice JH Mao1, KA Lindsay2, A Balmain3 and TE Wheldon1'4 'Department of Radiation Oncology, University of Glasgow, CRC Beatson Laboratories, Garscube Estate, Glasgow G61 1 BD, UK; 2Department of Mathematics, University of Glasgow, University Gardens, Glasgow, UK; 3CRC Department of Medical Oncology, University of Glasgow, CRC Beatson Laboratories, Garscube Estate, Glasgow G61 1 BD, UK; 4Department of Clinical Physics, University of Glasgow and West Glasgow Hospitals University NHS Trust, Western Infirmary, Glasgow G 1 6NT, UK Summary Stochastic models of tumorigenesis have been developed to investigate the implications of experimental data on tumour induction in wild-type and p53-deficient mice for tumorigenesis mechanisms. Conventional multistage models in which inactivation of each p53 allele represents a distinct stage predict excessively large numbers of tumours in p53-deficient genotypes, allowing this category of model to be rejected. Multistage multipath models, in which a p53-mediated pathway co-exists with one or more p53-independent pathways, are consistent with the data, although these models require unknown pathways and do not enable age-specific curves of tumour appearance to be computed. An alternative model that fits the data is the 'multigate' model in which tumorigenesis results from a small number of gate-pass (enabling) events independently of p53 status. The role of p53 inactivation is as a rate modifier that accelerates the gate-pass events. This model implies that wild-type p53 acts as a 'caretaker' to maintain genetic uniformity in cell populations, and that p53 inactivation increases the probability of occurrence of a viable cellular mutant by a factor of about ten. -
Bccipsuppresses Tumor Initiation but Is Required For
Published OnlineFirst October 21, 2013; DOI: 10.1158/0008-5472.CAN-13-1766 Cancer Tumor and Stem Cell Biology Research BCCIP Suppresses Tumor Initiation but Is Required for Tumor Progression Yi-Yuan Huang, Li Dai, Dakim Gaines, Roberto Droz-Rosario, Huimei Lu, Jingmei Liu, and Zhiyuan Shen Abstract Dysfunctions of genome caretaker genes contribute to genomic instability and tumor initiation. Because many of the caretaker genes are also essential for cell viability, permanent loss of function of these genes would prohibit further tumor progression. How essential caretaker genes contribute to tumorigenesis is not fully understood. Here, we report a "hit-and-run" mode of action for an essential caretaker gene in tumorigenesis. Using a BRCA2- interacting protein BCCIP as the platform, we found that a conditional BCCIP knockdown and concomitant p53 deletion caused rapid development of medulloblastomas, which bear a wide spectrum of alterations involving the Sonic Hedgehog (Shh) pathway, consistent with a caretaker responsibility of BCCIP on genomic integrity. Surprisingly, the progressed tumors have spontaneously lost the transgenic BCCIP knockdown cassette and restored BCCIP expression. Thus, a transient downregulation of BCCIP, but not necessarily a permanent mutation, is sufficient to initiate tumorigenesis. After the malignant transformation has been accomplished and autonomous cancer growth has been established, BCCIP reverses its role from a tumor-initiation suppressor to become a requisite for progression. This exemplifies a new type of tumor suppressor, which is distinct from the classical tumor suppressors that are often permanently abrogated during tumorigenesis. It has major implica- tions on how a nonmutagenic or transient regulation of essential caretaker gene contributes to tumorigenesis. -
Onc19 8REV 2..2
Oncogene (2000) 19, 1059 ± 1064 ã 2000 Macmillan Publishers Ltd All rights reserved 0950 ± 9232/00 $15.00 www.nature.com/onc Role of the tumor suppressor gene Brca1 in genetic stability and mammary gland tumor formation Chu-Xia Deng*,1 and Frank Scott1 1Genetics of Development and Disease Branch, 10/9N105, National Institute of Diabetes, Digestive and Kidney Diseases, National Institutes of Health, Bethesda, Maryland, MD 20892, USA Germline mutations in the tumor suppressor BRCA1 The BRCA1 gene contains 24 exons, which encode a predispose women to breast and ovarian cancers. Current large protein of 1863 amino acids in humans and 1812 evidence demonstrates that mutations in BRCA1 do not amino acids in mice (Bennett et al., 1995; Lane et al., directly result in tumor formation, but instead cause 1995; Marquis et al., 1995; Miki et al., 1994). It also genetic instability, subjecting cells to high risks of contains a number of motifs that may have distinct malignant transformation. In an animal model in which functions [reviewed in (Paterson, 1998), and Figure 1]. Brca1 is mutated speci®cally in mammary epithelium, The zinc ®nger domain in the amino terminus interacts tumorigenesis occurs in mutant glands at low frequency with DNA directly or indirectly through protein ± after a long latency. Notably, introduction of a p53-null protein interactions (Jin et al., 1997; Wu et al., 1996). allele signi®cantly enhanced mammary gland tumor The carboxyl terminus contains a BRCT domain, formation in Brca1 conditional mutant mice. These which is a relatively common feature of proteins results are consistent with a model that Brca1 is a involved in DNA damage response checkpoints (Bork caretaker gene, whose absence causes genetic instability et al., 1997). -
Bccipsuppresses Tumor Initiation but Is Required for Tumor Progression
Published OnlineFirst October 21, 2013; DOI: 10.1158/0008-5472.CAN-13-1766 Cancer Tumor and Stem Cell Biology Research BCCIP Suppresses Tumor Initiation but Is Required for Tumor Progression Yi-Yuan Huang, Li Dai, Dakim Gaines, Roberto Droz-Rosario, Huimei Lu, Jingmei Liu, and Zhiyuan Shen Abstract Dysfunctions of genome caretaker genes contribute to genomic instability and tumor initiation. Because many of the caretaker genes are also essential for cell viability, permanent loss of function of these genes would prohibit further tumor progression. How essential caretaker genes contribute to tumorigenesis is not fully understood. Here, we report a "hit-and-run" mode of action for an essential caretaker gene in tumorigenesis. Using a BRCA2- interacting protein BCCIP as the platform, we found that a conditional BCCIP knockdown and concomitant p53 deletion caused rapid development of medulloblastomas, which bear a wide spectrum of alterations involving the Sonic Hedgehog (Shh) pathway, consistent with a caretaker responsibility of BCCIP on genomic integrity. Surprisingly, the progressed tumors have spontaneously lost the transgenic BCCIP knockdown cassette and restored BCCIP expression. Thus, a transient downregulation of BCCIP, but not necessarily a permanent mutation, is sufficient to initiate tumorigenesis. After the malignant transformation has been accomplished and autonomous cancer growth has been established, BCCIP reverses its role from a tumor-initiation suppressor to become a requisite for progression. This exemplifies a new type of tumor suppressor, which is distinct from the classical tumor suppressors that are often permanently abrogated during tumorigenesis. It has major implica- tions on how a nonmutagenic or transient regulation of essential caretaker gene contributes to tumorigenesis. -
The Relationship Between Aging and Carcinogenesis: a Critical Appraisal
Critical Reviews in Oncology/Hematology 45 (2003) 277Á/304 www.elsevier.com/locate/critrevonc The relationship between aging and carcinogenesis: a critical appraisal Vladimir N. Anisimov * Department of Carcinogenesis and Oncogerontology, N.N. Petrov Research Institute of Oncology, Pesochny-2, 68 Leningradskaya St., St. Petersburg 197758, Russia Accepted 11 September 2002 Contents 1. Introduction ............................................. 278 2. Age-related increase in spontaneous tumor development . ................. 278 3. Is the susceptibility to carcinogenesis similar in different tissues at different age ....... 279 4. Aging and multi-stage carcinogenesis ............................... 280 5. Cellular senescence and carcinogenesis .............................. 284 6. Do carcinogens accelerate aging .................................. 286 7. Do premature aging promote carcinogenesis ........................... 289 8. Effect of genetic modifications of aging on carcinogenesis . ................. 290 9. Oxidative stress in aging and carcinogenesis ........................... 293 10. Life span extension and risk of cancer .............................. 293 11. Conclusions ............................................. 297 12. Reviewers .............................................. 297 Acknowledgements ............................................ 297 References ................................................. 297 Biography ................................................. 304 Abstract The incidence of cancer increases with age in -
A Short Review of the Genes Involved in the Development and Progression of Colorectal Cancer
BIOCELL Tech Science Press 2021 45(3): 483-487 A short review of the genes involved in the development and progression of colorectal cancer AMAYNA ZAKARIA; SYED ABDULLAH IBN ASADUZZAMAN; ZOBAYDA NAHAR; HAFSA JARIN SNIGDHA; TASKINA MURSHED; RASHED NOOR* Department of Life Sciences, School of Environment and Life Sciences, Independent University, Bangladesh (IUB), Dhaka, 1229, Bangladesh Key words: Colorectal cancer, Genes, Signal transduction pathways Abstract: The extent and aggression of colorectal cancer is a worldwide public health threat. Extensive research has been conducted on the pre-requisites leading to this fatal cancer. An array of genes along with their mutations and the signal transduction pathways leading to the cellular transformation into the cancerous cells have been investigated. Based on the knowledge gained so far, present review shortly discussed the role of the major genes especially those are involved in instigating abnormalities in the cellular cycles, cellular proliferation and differentiation. A simple but novel molecular scheme of the colorectal cancer development has also been plotted. Introduction within the right colon may trigger CRC (Medina Pabón and Babiker, 2020). On the contrary, in the case of HPCC-FAP, Defects in genomic organization have been linked to the hundreds or thousands of precancerous adenomatous polyps tumor progression have been suggested (defects in the form that, in turn, instigate the CRC (Medina Pabón and mitotic spindle leading to the formation of malignant Babiker, 2020). tumors) more -
The Expression and the Nuclear Activity of the Caretaker Gene Ku86 Are Modulated by Somatostatin
ORIGINAL PAPER The expression and the nuclear activity of the caretaker gene ku86 are modulated by somatostatin S. Pucci,1 E. Bonanno,1 F. Pichiorri,1 P. Mazzarelli,2 L.G. Spagnoli1 1Dept. of Biopathology, University of Rome “Tor Vergata”, Rome; 2Dept. of Dermatology, University of Naples ”Federico II”, Naples, Italy omatostatin (SST) is a peptide hormone, ©2004, European Journal of Histochemistry which plays an important regulatory role in Somatostatin is a peptide hormone that exerts antisecretory Sthe endocrine, paracrine and autocrine secre- and antiproliferative activities on some human tumors. The tions of several anterior pituitary, and gastrointesti- Ku70/86 heterodimer acts as regulatory subunit of the DNA dependent protein kinase and its DNA binding activity medi- nal hormones. ates DNA double strands breaks repair that is crucial to Besides, it has been shown that SST exerts an maintain the genetic integrity of the genome. The activation antiproliferative activity on some human cancer of the heterodimer regulates cell cycle progression and the activity of nuclear transcription factors involved in DNA repli- including breast cancer,pancreatic cancer,small cell cation and cell proliferation. Moreover Ku86 behaves as a lung carcinoma and neuroblastoma (De Vita et al., receptor for the growth inhibitory tetradecapeptide, somato- 1997). The biological activity of this peptide is statin. Herein we report that somatostatin treatment to a dependent on its interaction with specific receptors, colon carcinoma cell line (Caco-2) inhibits cell growth and, at same time, strongly modulates the activation of Ku70/86 whose structures have recently been elucidated by heterodimer and the levels of Ku86 in the nucleus by means of cloning experiments (Yamada et al., increasing its specific mRNA level. -
Multistage Carcinogenesis and the Incidence of Colorectal Cancer
Multistage carcinogenesis and the incidence of colorectal cancer E. Georg Luebeck* and Suresh H. Moolgavkar Fred Hutchinson Cancer Research Center, 1100 Fairview Avenue North, P.O. Box 19024, Seattle, WA 98109-1024 Edited by Alfred G. Knudson, Jr., Institute for Cancer Research, Philadelphia, PA, and approved September 11, 2002 (received for review February 27, 2002) We use general multistage models to fit the age-specific incidence ACF, also referred to as adenomatous crypts or microadenoma, of colorectal cancers in the Surveillance, Epidemiology, and End frequently show loss of heterozygosity on 5q, the locus of the Results registry, which covers Ϸ10% of the U.S. population, while adenomatous polyposis coli (APC) gene (14–17). ACF are simultaneously adjusting for birth cohort and calendar year effects. believed to be precursors to the adenomatous polyps, which in The incidence of colorectal cancers in the Surveillance, Epidemiol- turn are widely believed to be precursor lesions for colon ogy, and End Results registry is most consistent with a model carcinoma. The transition from adenoma to high-grade dysplasia positing two rare events followed by a high-frequency event in the (HGD) appears to involve the TP53 gene, considered a guardian conversion of a normal stem cell into an initiated cell that expands of the genome (12). Once HGD occurs, it has been suggested clonally to give rise to an adenomatous polyp. Only one more rare that ‘‘genetic chaos’’ ensues, setting the stage for malignant event appears to be necessary for malignant transformation. The transformation (18). two rare events involved in initiation are interpreted to represent Important insights into age effects and temporal trends in the homozygous loss of adenomatous polyposis coli gene function. -
BRCA1 Induces Antioxidant Gene Expression and Resistance to Oxidative Stress
[CANCER RESEARCH 64, 7893–7909, November 1, 2004] BRCA1 Induces Antioxidant Gene Expression and Resistance to Oxidative Stress Insoo Bae,1 Saijun Fan,1 Qinghui Meng,1 Jeong Keun Rih,1 Hee Jong Kim,1 Hyo Jin Kang,1 Jingwen Xu,1 Itzhak D. Goldberg,2 Anil K. Jaiswal,3 and Eliot M. Rosen1 1Department of Oncology, Lombardi Cancer Center, Georgetown University, Washington, DC; 2Department of Radiation Oncology, Long Island Jewish Medical Center, The Long Island Campus for the Albert Einstein College of Medicine, New Hyde Park, New York; and 3Department of Pharmacology, Baylor College of Medicine, Houston, Texas ABSTRACT binding protein), retinoblastoma 1, retinoblastoma 1-associated pro- teins (RbAp46/48), and several histone deaceylases (HDAC-1/2)], Mutations of the breast cancer susceptibility gene 1 (BRCA1), a tumor and/or sequence-specific DNA-binding transcription factors (e.g., suppressor, confer an increased risk for breast, ovarian, and prostate p53, c-Myc, estrogen receptor, and other proteins; refs. 7–12). cancers. To investigate the function of the BRCA1 gene, we performed DNA microarray and confirmatory reverse transcription-PCR analyses to Some of the functions of BRCA1 cited above may be due, in part, identify BRCA1-regulated gene expression changes. We found that to regulation of specific transcriptional pathways by BRCA1, but the BRCA1 up-regulates the expression of multiple genes involved in the linkage of these functions to BRCA1-regulated transcription is not cytoprotective antioxidant response, including glutathione S-transferases, well understood. We used cell culture models of BRCA1 overexpres- oxidoreductases, and other antioxidant genes. Consistent with these find- sion, underexpression, and mutational inactivation to identify patterns ings, BRCA1 overexpression conferred resistance while BRCA1 deficiency of BRCA1-regulated gene expression.