Chirality, Gelation Ability and Crystal Structure: Together Or Apart? Alkyl Phenyl Ethers of Glycerol As Simple Lmwgs
Total Page:16
File Type:pdf, Size:1020Kb
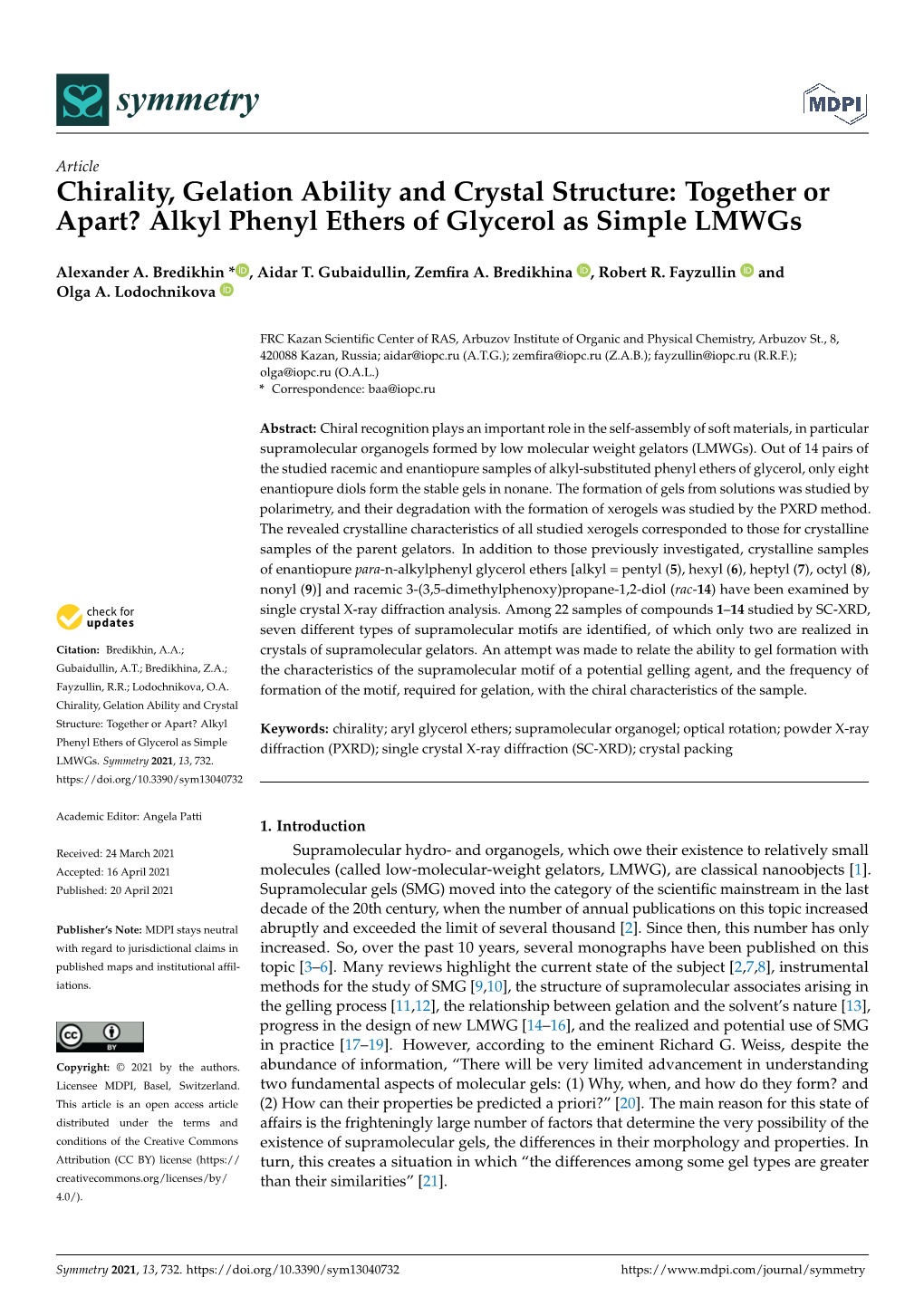
Load more
Recommended publications
-
Sensors 2010, 10, 6796-6820; Doi:10.3390/S100706796 OPEN ACCESS Sensors ISSN 1424-8220
Sensors 2010, 10, 6796-6820; doi:10.3390/s100706796 OPEN ACCESS sensors ISSN 1424-8220 www.mdpi.com/journal/sensors Review Intelligent Chiral Sensing Based on Supramolecular and Interfacial Concepts Katsuhiko Ariga 1,2,*, Gary J. Richards 1, Shinsuke Ishihara 1, Hironori Izawa 1,2 and Jonathan P. Hill 1,2 1 World Premier International (WPI) Research Center for Materials Nanoarchitectonics (MANA), National Institute for Materials Science (NIMS), 1-1 Namiki, Tsukuba 305-0044, Japan 2 Japan Science and Technology Agency, CREST, 1-1 Namiki, Tsukuba 305-0044, Japan * Author to whom correspondence should be addressed; E-Mail: [email protected]; Tel.: +81-29-860-4597; Fax: +81-29-860-4832. Received: 17 June 2010; in revised form: 7 July 2010 / Accepted: 8 July 2010 / Published: 13 July 2010 Abstract: Of the known intelligently-operating systems, the majority can undoubtedly be classed as being of biological origin. One of the notable differences between biological and artificial systems is the important fact that biological materials consist mostly of chiral molecules. While most biochemical processes routinely discriminate chiral molecules, differentiation between chiral molecules in artificial systems is currently one of the challenging subjects in the field of molecular recognition. Therefore, one of the important challenges for intelligent man-made sensors is to prepare a sensing system that can discriminate chiral molecules. Because intermolecular interactions and detection at surfaces are respectively parts of supramolecular chemistry -
Chiral Metal–Macrocycle Frameworks: Supramolecular Chirality Induction and Helicity Cite This: Chem
Chemical Science View Article Online EDGE ARTICLE View Journal | View Issue Chiral metal–macrocycle frameworks: supramolecular chirality induction and helicity Cite this: Chem. Sci.,2016,7, 2217 inversion of the helical macrocyclic structures† Ryou Kubota, Shohei Tashiro and Mitsuhiko Shionoya* Porous molecular solids composed of discrete macrocycles/cages have great potential for catalysis, separation and sensing techniques. Dynamic structural transformation of the host building blocks, especially a helicity inversion responsive to chemical triggers, is central to upgrading the spatial functions. Here we have achieved the syntheses of homochiral porous molecular solids composed of helical metal macrocycles through supramolecular chirality induction to both enantiomorphic forms with Received 27th November 2015 the aid of two different enantiopure sugar-derived lactones in the crystallization process. Moreover, we Accepted 23rd December 2015 found that the helicity of the macrocyclic skeletons can be inverted in the crystalline state only by DOI: 10.1039/c5sc04570c changing the type of solvent. This finding would lead to dynamic control of space chirality in connection Creative Commons Attribution 3.0 Unported Licence. www.rsc.org/chemicalscience with optical resolution, chiral amplification and asymmetric reactions. Introduction ions normally affords racemic crystals containing an equal amount of (M)- and (P)-forms of helical metal–macrocycles. Porous molecular solids (PMSs) composed of shape-persistent Moreover, it is difficult to regulate asymmetric crystallization macrocycles/cages show promise for solid-state functions from an equilibrated mixture of helical macrocycles in such as molecular storage, separation and catalysis.1,2 a predictable manner, and it is even more challenging to Through weak intermolecular interactionssuchasvander control the chirality of porous crystals in the solid state. -
Supramolecular Chirality: Solvent Chirality Transfer in Molecular Chemistry and Polymer Chemistry
Symmetry 2014, 6, 677-703; doi:10.3390/sym6030677 OPEN ACCESS symmetry ISSN 2073-8994 www.mdpi.com/journal/symmetry Review Supramolecular Chirality: Solvent Chirality Transfer in Molecular Chemistry and Polymer Chemistry Michiya Fujiki Graduate School of Materials Science, Nara Institute of Science and Technology (NAIST), 8916-5 Takayama, Ikoma, Nara 630-0036, Japan; E-Mail: [email protected]; Tel.: +81-743-72-6040 Received: 15 July 2014; in revised form: 7 August 2014 / Accepted: 7 August 2014 / Published: 13 August 2014 Abstract: Controlled mirror symmetry breaking arising from chemical and physical origin is currently one of the hottest issues in the field of supramolecular chirality. The dynamic twisting abilities of solvent molecules are often ignored and unknown, although the targeted molecules and polymers in a fluid solution are surrounded by solvent molecules. We should pay more attention to the facts that mostly all of the chemical and physical properties of these molecules and polymers in the ground and photoexcited states are significantly influenced by the surrounding solvent molecules with much conformational freedom through non-covalent supramolecular interactions between these substances and solvent molecules. This review highlights a series of studies that include: (i) historical background, covering chiral NaClO3 crystallization in the presence of D-sugars in the late 19th century; (ii) early solvent chirality effects for optically inactive chromophores/fluorophores in the 1960s–1980s; and (iii) the recent development of mirror symmetry breaking from the corresponding achiral or optically inactive molecules and polymers with the help of molecular chirality as the solvent use quantity. Keywords: optically active; chiral; achiral; supramolecules; polymers; solvent; circular dichroism; circularly polarized luminescence; homochirality; molecular chirality 1. -
Chiral Solvation Induced Supramolecular Chiral Assembly of Achiral Polymers
Chapter 4 Chiral Solvation Induced Supramolecular Chiral Assembly of Achiral Polymers Wei Zhang, Yin Zhao and Lu Yin Additional information is available at the end of the chapter http://dx.doi.org/10.5772/67700 Abstract To date, liquid crystal chirality, mechanophysical chirality, circularly polarized photon chirality, gelation and chiral solvation are all feasible candidates to generate optically active polymers and supramolecular chirality when employing achiral molecules as start‐ ing substances. Among this, chiral‐solvation‐induced chirality is one of the dominant methods for construction of chirality from achiral sources, such as achiral poly(n‐hexyl isocyanate) (PHIC), π‐conjugated polymers, oligo(p‐phenylenevinylene), polyacetylenes, σ‐conjugated polysilanes and side‐chain polymers. Supramolecular chirality is well established through their intra‐ or inter‐molecular noncovalent interactions, such as van der Waals, CH/π, dipole‐dipole interactions, hydrogen bonding and metal‐ligand coordi‐ nating interactions. Compared with the traditional methods, this strategy avoids the use of expensive chiral reagents and also expands the scope towards challenging substrates. This chapter highlights a series of studies that include: (i) the development‐historical background of chiral solvent induction strategy; (ii) the chiral‐solvation‐induced chirality in small molecules and oligomers; and (iii) recent developments in polymers, especially in π‐conjugated polymers and σ‐conjugated polymers. Keywords: optical activity, supramolecular chirality, chiral solvation, self‐assembly, circular dichroism, circularly polarized luminescence 1. Introduction As early as the second half of the nineteenth century, many scientists have long thought that the intrinsic biomolecular homochirality found in the living world is the origin of life on earth, since inherent optical activity exists inside all living organisms [1–14]. -
Chirality in Supramolecular Assemblies Chirality in Supramolecular Assemblies
Chirality in Supramolecular Assemblies Chirality in Supramolecular Assemblies Causes and Consequences Edited by F. RICHARD KEENE Department of Chemistry, School of Physical Sciences, University ofAdelaide, Australia WILEY Tbis edition first published 2017 © 2017 John Wiley & Sons, Ltd Registered Office John Wiley & Sons, Ltd, TbeAtrium, Southern Gate, Chichester, West Sussex, P019 8SQ, United Kingdom For details of our global editorial offices, for customer services and for information about how to apply for permission to reuse the copyright material in this book please see our website atwww.wiley.com. The right of the author to be identified as the author of this work has been asserted in accordance with the Copyright, Designs and Patents Act 1988. All rights reserved. No part of this publication may be reproduced, stored in a retrieval system, or transmitted, in any form or by any means, electronic, mechanical, photocopying, recording or otherwise, except as permitted by the UK Copyright, Designs and Patents Act 1988, without the prior permission of the publisher. Wiley also publishes its books in a variety of electronic formats. Some content that appears in p1int may not be available in electronic books. Designations used by companies to distinguish their products are often claimed as trademarks. All brand names and product names used in this book are trade names, service marks, trademarks or registered trademarks of their respective owners. The publisher is not associated with any product or vendor mentioned in this book. Limit of Liability/Disclaimer of Warranty: While the publisher and author have used their best efforts in preparing this book, they make no representations or warranties with respect to the accuracy or completeness of the contents of this book and specifically disclaim any implied warranties of merchantability or fitness for a particular purpose. -
Supramolecular Chirality of Hydrogen-Bonded Rosette Assemblies Mercedes Crego-Calama
Supramolecular chirality of hydrogen-bonded rosette assemblies Mercedes Crego-Calama To cite this version: Mercedes Crego-Calama. Supramolecular chirality of hydrogen-bonded rosette assemblies. Supramolecular Chemistry, Taylor & Francis: STM, Behavioural Science and Public Health Titles, 2007, 19 (01-02), pp.95-106. 10.1080/10610270600981716. hal-00513491 HAL Id: hal-00513491 https://hal.archives-ouvertes.fr/hal-00513491 Submitted on 1 Sep 2010 HAL is a multi-disciplinary open access L’archive ouverte pluridisciplinaire HAL, est archive for the deposit and dissemination of sci- destinée au dépôt et à la diffusion de documents entific research documents, whether they are pub- scientifiques de niveau recherche, publiés ou non, lished or not. The documents may come from émanant des établissements d’enseignement et de teaching and research institutions in France or recherche français ou étrangers, des laboratoires abroad, or from public or private research centers. publics ou privés. Supramolecular Chemistry For Peer Review Only Supramolecular chirality of hydrogen-bonded rosette assemblies Journal: Supramolecular Chemistry Manuscript ID: GSCH-2006-0028 Manuscript Type: Review Date Submitted by the 31-May-2006 Author: Complete List of Authors: crego-calama, mercedes; University of Twente, SMCT Supramolecular chirality, noncovalent synthesis, amplification of Keywords: chirality, diastereomeric synthesis, enantiomeric synthesis Note: The following files were submitted by the author for peer review, but cannot be converted to PDF. You must view these files (e.g. movies) online. tif.sit URL: http:/mc.manuscriptcentral.com/tandf/gsch Email: [email protected] Page 1 of 20 Supramolecular Chemistry 1 2 3 4 5 Supramolecular chirality of hydrogen-bonded 6 7 rosette assemblies 8 9 10 Socorro Vázquez-Campos, Mercedes Crego-Calama*, and David N. -
Supramolecular Stereochemistry NATO ASI Series Advanced Science Institutes Series
Supramolecular Stereochemistry NATO ASI Series Advanced Science Institutes Series A Series presenting the results of activities sponsored by the NATO Science Committee, which aims at the dissemination of advanced scientific and technological knowledge, with a view to strengthening links between scientific communities. The Series is published by an international board of publishers in conjunction with the NATO Scientific Affairs Division A Life Sciences Plenum Publishing Corporation B Physics London and New York C Mathematical and Physical Sciences Kluwer Academic Publishers D Behavioural and Social Sciences Dordrecht, Boston and London E Applied Sciences F Computer and Systems Sciences Springer-Verlag G Ecological Sciences Berlin, Heidelberg, New York, London, H Cell Biology Paris and Tokyo I Global Environmental Change PARTNERSHIP SUB-SERIES 1. Disarmament Technologies Kluwer Academic Publishers 2. Environment Springer-Verlag / Kluwer Academic Publishers 3. High Technology Kluwer Academic Publishers 4. Science and Technology Policy Kluwer Academic Publishers 5. Computer Networking Kluwer Academic Publishers The Partnership Sub-Series incorporates activities undertaken in collaboration with NATO's Cooperation Partners, the countries of the CIS and Central and Eastern Europe, in Priority Areas of concern to those countries. NATO-PCO-DATA BASE The electronic index to the NATO ASI Series provides full bibliographical references (with keywords and/or abstracts) to more than 50000 contributions from international scientists published in all sections of the NATO ASI Series. Access to the NATO-PCO-DATA BASE is possible in two ways: - via online FILE 128 (NATO-PCO-DATA BASE) hosted by ESRIN, Via Galileo Galilei, I-00044 Frascati, Italy. - via CD-ROM "NATO-PCO-DATA BASE" with user-friendly retrieval software in English, French and German (© WTV GmbH and DATAWARE Technologies Inc. -
31St International Symposium on Chirality
31st International Symposium on Chirality 14th - 17th July 2019 Bordeaux, France Chirality 2019 Programme Programme WELCOME TO BORDEAUX It is our great pleasure to welcome you to Bordeaux for the 31st International CONTENT Symposium on Chirality - CHIRALITY 2019 held at ENSEIRB MATMECA, located on the campus of the University of Bordeaux, a short tram ride away from the centre of Bordeaux―the “Port of the Moon”―and its UNESCO world heritage-protected, historic centre created in the Age of Enlightenment. This annual three and a half days event is dedicated to state-of-the-art research Welcome note page 3 in various fields of chirality, and was first organised in 1991. CHIRALITY 2019 in International committee page 4 Bordeaux follows the previous meetings held in Princeton (2018), Tokyo (2017), Local organising committee page 5 Heidelberg (2016), Boston (2015), and Prague (2014). Invited speakers page 6 Chirality Medal 2019 page 8 During this 2019 conference, achievements in enantioseparation from some of the Practical information page 10-11 founders of the CHIRALITY conference will be highlighted by invited speakers. Also, the annual Chirality Medal will be award to a researcher who has made significant Bordeaux general information page 12-13 contributions to this field over the course of his career. This year, the Chirality Medal Gala dinner page 14-15 winner is Professor Laurence A. Nafie from Syracuse University, USA. Partners & sponsors page 16 Programme This exciting programme brings together students, postdoc fellows and established Sunday 14th July page 17 researchers from academia, as well as industrialists, with topics covering the whole Monday 15th July page 18 to 21 spectrum of chirality research. -
PROGRAMME SUNDAY 14Th JULY
PROGRAMME SUNDAY 14th JULY Time Amphitheater F 9.30 Welcome coffee 10.00 Short course 1: Chiroptical spectroscopy methods for structural analysis of chiral molecules Teachers: Giovanna LONGHI, University of Brescia, Italy Prasad POLAVARAPU, Vanderbilt University, USA 12.30 Lunch 13.30 Short courses 2 : Materials Teachers: Anja PALMANS, Eindhoven University of Technology, The Netherlands Gil MARKOVICH, University of Tel Aviv, Israel Amphitheater 1 16.00 Opening ceremony Reiko ODA, Research director, Univ. of Bordeaux / CNRS / Bordeaux INP Jeanne CRASSOUS, Research director, University of Rennes / CNRS Jérôme LACOUR, Research director, University of Geneva, Switzerland Philippe MORETTO, Vice president for Research, University of Bordeaux Erick DUFOURC, Deputy Scientific Director, Institute of Chemistry (INC) Leon GHOSEZ, Emeritus Professor UCL, University of Bordeaux, France 16.30 Award lecture: Laurence A. NAFIE, Syracuse University, USA Vibrational optical activity: discovery, development and future challenges 17.45 Chirality medal ceremony 18.00 Welcome reception 20.00 End Plenary PROGRAMME Keynote MONDAY 15th JULY AM Oral presentation AM Time Amphitheater 1 Amphitheater 2 AM 8:45 Donald HILVERT, ETH Zurich, Switzerland Chair: 9:30 Design and evolution of artificial metalloenzymes K. Saigo Polymer / materials 1 Spectroscopy 1 Chair: E. Hillard Chair: C. Merten Eiji YASHIMA, Nagoya University, Japan 9:30 Giovanna LONGHI, University of Brescia, Italy Control of macromolecular helicity for the 9:55 Circularly polarized luminescence of twisted -
Chiral Recognition and Selection During the Self-Assembly Process of Protein-Mimic Macroanions
ARTICLE Received 6 May 2014 | Accepted 2 Feb 2015 | Published 10 Mar 2015 DOI: 10.1038/ncomms7475 Chiral recognition and selection during the self-assembly process of protein-mimic macroanions Panchao Yin1,2, Zhi-Ming Zhang3, Hongjin Lv4, Tao Li5, Fadi Haso1,2, Lang Hu1,2, Baofang Zhang1,2, John Bacsa4, Yongge Wei6, Yanqing Gao3, Yu Hou4, Yang-Guang Li3, Craig L. Hill4, En-Bo Wang3 & Tianbo Liu1,2 The research on chiral recognition and chiral selection is not only fundamental in resolving the puzzle of homochirality, but also instructive in chiral separation and stereoselective catalysis. Here we report the chiral recognition and chiral selection during the self-assembly process of two enantiomeric wheel-shaped macroanions, [Fe28(m3-O)8(Tart)16 20 À (HCOO)24] (Tart ¼ D-orL-tartaric acid tetra-anion). The enantiomers are observed to remain self-sorted and self-assemble into their individual assemblies in their racemic mixture solution. The addition of chiral co-anions can selectively suppress the self-assembly process of the enantiomeric macroanions, which is further used to separate the two enantiomers from their mixtures on the basis of the size difference between the monomers and the assemblies. We believe that delicate long-range electrostatic interactions could be responsible for such high-level chiral recognition and selection. 1 Department of Polymer Science, The University of Akron, Akron, Ohio 44325-3909, USA. 2 Department of Chemistry, Lehigh University, Bethlehem, Pennsylvania 18015, USA. 3 Key Laboratory of Polyoxometalate Science of Ministry of Education, Northeast Normal University, Changchun, Jilin 130024, China. 4 Department of Chemistry, Emory University, Atlanta, Georgia, 30322, USA. -
Induction and Control of Supramolecular Chirality by Light in Self-Assembled Helical Nanostructures
ARTICLE Received 7 Oct 2014 | Accepted 18 Mar 2015 | Published 23 Apr 2015 DOI: 10.1038/ncomms7959 Induction and control of supramolecular chirality by light in self-assembled helical nanostructures Jisung Kim1, Jinhee Lee1, Woo Young Kim2, Hyungjun Kim1, Sanghwa Lee1, Hee Chul Lee3, Yoon Sup Lee1, Myungeun Seo4 & Sang Youl Kim1 Evolution of supramolecular chirality from self-assembly of achiral compounds and control over its handedness is closely related to the evolution of life and development of supramolecular materials with desired handedness. Here we report a system where the entire process of induction, control and locking of supramolecular chirality can be manipulated by light. Combination of triphenylamine and diacetylene moieties in the molecular structure allows photoinduced self-assembly of the molecule into helical aggregates in a chlorinated solvent by visible light and covalent fixation of the aggregate via photopolymerization by ultraviolet light, respectively. By using visible circularly polarized light, the supramolecular chirality of the resulting aggregates is selectively and reversibly controlled by its rotational direction, and the desired supramolecular chirality can be arrested by irradiation with ultraviolet circularly polarized light. This methodology opens a route to ward the formation of supramolecular chiral conducting nanostructures from the self-assembly of achiral molecules. 1 Department of Chemistry, Korea Advanced Institute of Science and Technology (KAIST), 291 Daehak-ro, Yuseong-gu, Daejeon 305-701, Korea. 2 Department of Mechanical Engineering, KAIST, Daejeon 305-701, Korea. 3 Department of Electronic Engineering, KAIST, Daejeon 305-701, Korea. 4 Graduate School of Nanoscience and Technology, KAIST, Daejeon 305-701, Korea. Correspondence and requests for materials should be addressed to M.S. -
Impact of Chirality on Hydrogen-Bonded Supramolecular
Impact of chirality on hydrogen-bonded supramolecular assemblies and photoconductivity of diketopyrrolopyrrole derivatives Swann Militzer, Nozomi Nishimura, Nelson Ricardo Ávila-Rovelo, Wakana Matsuda, Duncan Schwaller, Philippe Mesini, Shu Seki, Amparo Ruiz Carretero To cite this version: Swann Militzer, Nozomi Nishimura, Nelson Ricardo Ávila-Rovelo, Wakana Matsuda, Duncan Schwaller, et al.. Impact of chirality on hydrogen-bonded supramolecular assemblies and photocon- ductivity of diketopyrrolopyrrole derivatives. Chemistry - A European Journal, Wiley-VCH Verlag, 2010, 10.1002/chem.202001540. hal-02868137 HAL Id: hal-02868137 https://hal.archives-ouvertes.fr/hal-02868137 Submitted on 8 Jan 2021 HAL is a multi-disciplinary open access L’archive ouverte pluridisciplinaire HAL, est archive for the deposit and dissemination of sci- destinée au dépôt et à la diffusion de documents entific research documents, whether they are pub- scientifiques de niveau recherche, publiés ou non, lished or not. The documents may come from émanant des établissements d’enseignement et de teaching and research institutions in France or recherche français ou étrangers, des laboratoires abroad, or from public or private research centers. publics ou privés. Impact of chirality on hydrogen-bonded supramolecular assemblies and photoconductivity of diketopyrrolopyrrole derivatives Swann Militzer,[a] Nozomi Nishimura, [b] Nelson Ricardo Ávila-Rovelo,[a] Wakana Matsuda, [b] Duncan Schwaller, [a] Philippe J. Mésini,[a] Shu Seki,[b] and Amparo Ruiz-Carretero*[a] [a] S. Militzer, N. R. Ávila-Rovelo, D. Schwaller, P. J. Mésini, A. Ruiz-Carretero University of Strasbourg, Institute Charles Sadron, CNRS, UPR22 23 Rue du Loess, 67000 Strasbourg Cedex 2, France E-mail: [email protected] [b] N.