Mineral Formation on Metallic Copper in Afuture Repository Site Environment
Total Page:16
File Type:pdf, Size:1020Kb
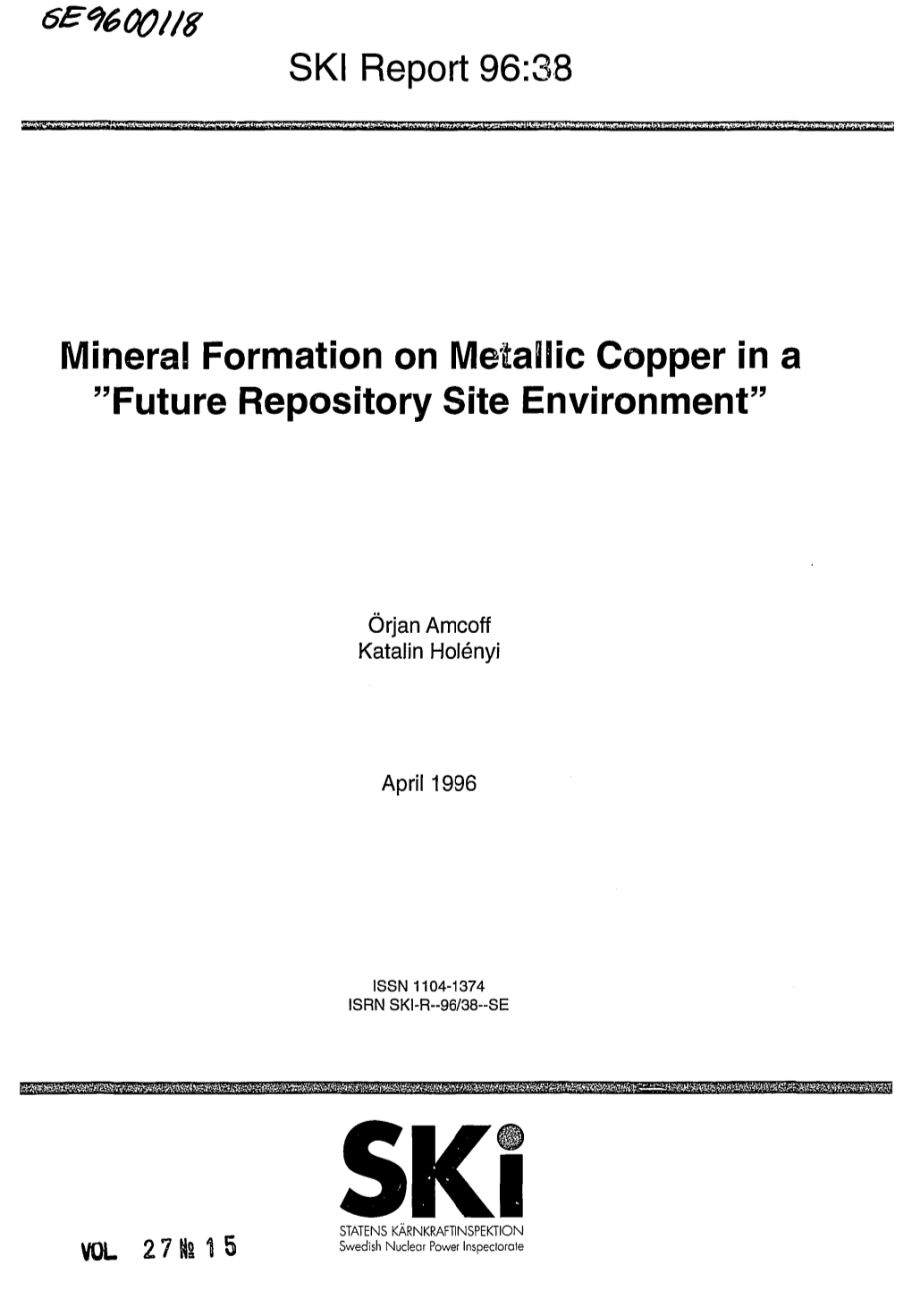
Load more
Recommended publications
-
Paolovite Pd2sn C 2001-2005 Mineral Data Publishing, Version 1
Paolovite Pd2Sn c 2001-2005 Mineral Data Publishing, version 1 Crystal Data: Orthorhombic. Point Group: 2/m 2/m 2/m. As irregular grains embedded in other minerals. Twinning: Polysynthetic. Physical Properties: Hardness = n.d. VHN = 329–378 (10 g load). D(meas.) = n.d. D(calc.) = 11.08 Optical Properties: Opaque. Color: Lilac-rose. Luster: Metallic. Pleochroism: Dark lilac-rose to pale rose. R1–R2: (400) — , (420) 44.4–46.5, (440) 44.6–47.2, (460) 45.0–48.2, (480) 45.5–49.1, (500) 45.9–50.3, (520) 46.7–51.6, (540) 47.7–52.8, (560) 49.0–54.1, (580) 50.9–55.4, (600) 52.9–56.7, (620) 55.3–57.7, (640) 57.7–59.2, (660) 59.8–59.5, (680) 61.5–60.3, (700) 62.7–60.6 Cell Data: Space Group: P bnm. a = 8.11(1) b = 5.662(6) c = 4.324(2) Z = 4 X-ray Powder Pattern: Oktyabr deposit, Russia. 2.28 (100), 2.16 (70), 1.955 (50), 2.36 (40), 1.397 (40), 1.315 (40), 1.120 (40) Chemistry: (1) (2) (3) Pd 64.8 64.3 64.19 Pt 2.5 Sn 35.5 35.0 35.81 Sb 0.3 Bi 0.2 Total 103.3 99.3 100.00 (1) Oktyabr deposit, Russia; by electron microprobe, corresponding to (Pd1.98Pt0.04)Σ=2.02Sn0.98. (2) Western Platinum mine, South Africa; by electron microprobe, corresponding to Pd2.02Sn0.98. (3) Pd2Sn. Occurrence: In Cu–Ni sulfide ores; in cubanite–chalcopyrite, cubanite–talnakhite, and cubanite–mooihoekite assemblages (Oktyabr deposit, Russia). -
Podiform Chromite Deposits—Database and Grade and Tonnage Models
Podiform Chromite Deposits—Database and Grade and Tonnage Models Scientific Investigations Report 2012–5157 U.S. Department of the Interior U.S. Geological Survey COVER View of the abandoned Chrome Concentrating Company mill, opened in 1917, near the No. 5 chromite mine in Del Puerto Canyon, Stanislaus County, California (USGS photograph by Dan Mosier, 1972). Insets show (upper right) specimen of massive chromite ore from the Pillikin mine, El Dorado County, California, and (lower left) specimen showing disseminated layers of chromite in dunite from the No. 5 mine, Stanislaus County, California (USGS photographs by Dan Mosier, 2012). Podiform Chromite Deposits—Database and Grade and Tonnage Models By Dan L. Mosier, Donald A. Singer, Barry C. Moring, and John P. Galloway Scientific Investigations Report 2012-5157 U.S. Department of the Interior U.S. Geological Survey U.S. Department of the Interior KEN SALAZAR, Secretary U.S. Geological Survey Marcia K. McNutt, Director U.S. Geological Survey, Reston, Virginia: 2012 This report and any updates to it are available online at: http://pubs.usgs.gov/sir/2012/5157/ For more information on the USGS—the Federal source for science about the Earth, its natural and living resources, natural hazards, and the environment—visit http://www.usgs.gov or call 1–888–ASK–USGS For an overview of USGS information products, including maps, imagery, and publications, visit http://www.usgs.gov/pubprod To order this and other USGS information products, visit http://store.usgs.gov Suggested citation: Mosier, D.L., Singer, D.A., Moring, B.C., and Galloway, J.P., 2012, Podiform chromite deposits—database and grade and tonnage models: U.S. -
Cu-Fe-S Phases in Lunar Rocks
American Mineralogist, Volume 58, pages 952-954, 1973 Cu-Fe-SPhases in LunarRocks LawnnNcn A. Tevronl Departmentol Geosciences,Purdue Uniuersity, Lalaye t te, I ndiana47 907 KBNNrrn L. Wnrrnus Departmentof Applied Earth Sciences,Stanlord Uniaersity, Stanford, Calilornia 943 0 5 Abstract The sulfide minerals reported to be present in lunar rocks include troilite, mackinawite, the discredited mineral "chalcopyrrhotite", sphalerite, chalcopyrite, and cubanite. Apollo 12 sam- ple l2D2l,l34 contains these last two minerals, and the first chemical analyses of the Cu-Fe-S phases are presented. These minerals occur along cracks and grain boundaries within troilite and are probably exsolution products formed at low temperatures (i.e, 100"-300'C) from a cupriferous troilite. Introduction Cu-Fe-S Minerals The opaqueminerals, although constituting only a "Chalcopyrrhotite", a discreditedmineral (Yund minor amount of a given sample,have proven useful and Kullerud, 1966; Cabri, 7967) , hasbeen reported as indicators of the genesisand cooling histories of from Apollo 12, 14, and 15 rocks (El Goresyet al, the lunar rocks (e.9., Reid, 1971; Taylor and Mc- l97la,b; 1972a,b). It wasdescribed as a yellowish, Callister, 19721,Taylor et al, I973b). The most chalcopyrite-coloredphase which appearsisotropic abundant opaque mineral is ilmenite, and many of and is alwaysfound within troilite. It is probablethat the oxide phasesin lunar rocks are unique (e.6'., this phase is either talnakhite, a mineral originally armalcolite as per Anderson et al, l97A). In addi- describedby Cabri (1967) andhaving a composition tion to native Fe metal, native Cu, and schreibersite, of CusFesSro(Cabri and Harris, 1971) near chal- the remaining opaqueminerals consistof sulfides. -
Washington State Minerals Checklist
Division of Geology and Earth Resources MS 47007; Olympia, WA 98504-7007 Washington State 360-902-1450; 360-902-1785 fax E-mail: [email protected] Website: http://www.dnr.wa.gov/geology Minerals Checklist Note: Mineral names in parentheses are the preferred species names. Compiled by Raymond Lasmanis o Acanthite o Arsenopalladinite o Bustamite o Clinohumite o Enstatite o Harmotome o Actinolite o Arsenopyrite o Bytownite o Clinoptilolite o Epidesmine (Stilbite) o Hastingsite o Adularia o Arsenosulvanite (Plagioclase) o Clinozoisite o Epidote o Hausmannite (Orthoclase) o Arsenpolybasite o Cairngorm (Quartz) o Cobaltite o Epistilbite o Hedenbergite o Aegirine o Astrophyllite o Calamine o Cochromite o Epsomite o Hedleyite o Aenigmatite o Atacamite (Hemimorphite) o Coffinite o Erionite o Hematite o Aeschynite o Atokite o Calaverite o Columbite o Erythrite o Hemimorphite o Agardite-Y o Augite o Calciohilairite (Ferrocolumbite) o Euchroite o Hercynite o Agate (Quartz) o Aurostibite o Calcite, see also o Conichalcite o Euxenite o Hessite o Aguilarite o Austinite Manganocalcite o Connellite o Euxenite-Y o Heulandite o Aktashite o Onyx o Copiapite o o Autunite o Fairchildite Hexahydrite o Alabandite o Caledonite o Copper o o Awaruite o Famatinite Hibschite o Albite o Cancrinite o Copper-zinc o o Axinite group o Fayalite Hillebrandite o Algodonite o Carnelian (Quartz) o Coquandite o o Azurite o Feldspar group Hisingerite o Allanite o Cassiterite o Cordierite o o Barite o Ferberite Hongshiite o Allanite-Ce o Catapleiite o Corrensite o o Bastnäsite -
Ajoite: New Data
American Mineralogist, Volume 66, pages 201-203, 1981 Ajoite: new data GEORGE Y. CHAO Department of Geology, Carleton University Ottawa, Ontario Kl S 5B6, Canada Abstract New data show that ajoite is triclinic, PI or pI, a ==13.637, b ==14.507, c ==13.620A, a == 107.16, f3 = 105.45, y = 110.57°; Z ==3. The mineral is biaxial positive, 2V ==80°, a ==1.550, f3 = 1.583, y = 1.641 (in Na light); pleochroic: X = very light bluish green, Y -- Z ==brilliant bluish green. {010} cleavage is perfect. The orientation of the principal vibration directions is defined by the spherical coordinates X(26.5°, 80°), Y(118°, 79°), Z(-104.5°, 15°). The ex- tinction angle c: Z' on (0 I0) is 150. Electron microprobe and chemical analyses gave Si02 41.2, Al203 3.81, CuO 42.2, MnO 0.02, FeO 0.11, CaO 0.04, Na20 0.84, K20 2.50, H20 (TGA to 1000°C) 8.35, sum 99.07 wt.%. The analysis corresponds to (Ko.70NaO.36Cao.Ol)(CUt,.97 Feo.o2)Alo.98Si9.oo024(OH)6'3.09H20 or ideally, (K,Na)Cu7AISi9024(OH)6' 3H20. TGA showed a two-stage dehydration; 50% of the total water was released between 70° and 425°C and the rest between 4250 and 800°C. Half of the water is zeolitic in nature. Introduction are always present. The termination on c may be ei- Ajoite, first described by Schaller and Vlisidis ther {001} or {203} or both. (1958) from Ajo, Pima County, Arizona, was thought to be monoclinic on the basis of optical studies. -
Supergene Mineralogy and Processes in the San Xavier Mine Area, Pima County, Arizona
Supergene mineralogy and processes in the San Xavier mine area, Pima County, Arizona Item Type text; Thesis-Reproduction (electronic) Authors Arnold, Leavitt Clark, 1940- Publisher The University of Arizona. Rights Copyright © is held by the author. Digital access to this material is made possible by the University Libraries, University of Arizona. Further transmission, reproduction or presentation (such as public display or performance) of protected items is prohibited except with permission of the author. Download date 28/09/2021 18:44:48 Link to Item http://hdl.handle.net/10150/551760 SUPERGENE MINERALOGY AND PROCESSES IN THE SAN XAVIER MINE AREA— PIMA COUNTY, ARIZONA by L. Clark Arnold A Thesis Submitted to the Faculty of the DEPARTMENT OF GEOLOGY In Partial Fulfillment of the Requirements For the Degree of MASTER OF SCIENCE In the Graduate College THE UNIVERSITY OF ARIZONA 1964 STATEMENT BY AUTHOR This thesis has been submitted in partial fulfillment of require ments for an advanced degree at The University of Arizona and is de posited in the University Library to be made available to borrowers under rules of the Library. Brief quotations from this thesis are allowable without special permission, provided that accurate acknowledgment of source is made. Requests for permission for extended quotation from or reproduction of this manuscript in whole or in part may be granted by the head of the major department or the Dean of the Graduate College when in his judg ment the proposed use of the material is in the interests of scholarship. In all other instances, however, permission must be obtained from the author. -
Uranium in Situ Leaching
IAEA-TECDOC-720 Uranium in situ leaching Proceedings Technicala of Committee Meeting held in Vienna, 5-8 October 1992 INTERNATIONAL ATOMIC ENERGY AGENC\ /A Y The IAEA doe t normallsno y maintain stock f reportso thin si s series. However, microfiche copies of these reports can be obtained from IIMIS Clearinghouse International Atomic Energy Agency Wagramerstrasse5 P.O. Box 100 A-1400 Vienna, Austria Orders shoul accompaniee db prepaymeny db f Austriao t n Schillings 100,- in the form of a cheque or in the form of IAEA microfiche service coupons which may be ordered separately from the INIS Clearinghouse. Copies of this IAEA-TECDOC may be obtained from: Nuclear Material Fued san l Cycle Technology Section International Atomic Energy Agency Wagramerstrasse 5 P.O. Box 100 A-1400 Vienna, Austria URANIU SITMN I U LEACHING IAEA, VIENNA, 1993 IAEA-TECDOC-720 ISSN 1011-4289 Printe IAEe th AustriAn y i d b a September 1993 FOREWORD The IAEA's latest published estimates show that nuclear power production worldwide will maintain modest growth well into the next century. These estimates indicate that nuclear energy production will gro averagn wo 1.5y eb % 2.5o t year %pe r worldwide ove nex e decadeso rth tw t s i t I . for this reason that despite the continuing depressed uranium market, the question of uranium supply demand an d remain issun sa e that needaddressee b o st monitoredd dan developmentsw Ne . e du , to the recent entry of the Commonwealth of Independent States and China into the uranium market, increase such a need. -
Multianalytical Investigation and Conservation of Unique Copper Model Tools from Ancient Egyptian Dark Age
Ge-conservación Conservação | Conservation Multianalytical Investigation and Conservation of Unique Copper Model Tools from Ancient Egyptian Dark Age Manal Maher, Yussri Salem Abstract: The article presented multianalytical investigation of a unique set of copper model tools dated back to Dark Age, from the tomb of KHENNU AND APA-EM-SA-F (289) in the south of Memphis, Saqqara. Stereomicroscope was used to examine the morphology of outer surface corrosion products. Metallographic microscope was used to investigate the microstructure of the metal core and the stratigraphy of corrosion layers. SEM-EDX was used to identify the elemental composition of the objects. XRD and Raman spectroscopy were used to analyze the outer surface and the internal corrosion respectively. The microscopic investigation revealed the corrosion layers consists of external layer, under-surface layer and internal corrosion products. Cuprite, paratacamite, nantokite, atacamite, malachite and chalconatronite were identified by XRD and Raman spectroscopy as a surface and internal corrosion. SEM-EDX revealed that the case-study objects consist of copper metal without any further alloying elements. The study presented suitable treatment for these friable objects or such cases, and then presented a safe fixing procedure by a sewing technique via transparent inert threads. Keyword: Dark Age, copper model tools, first intermediate period, corrosion products, transparent inert threads Investigación multianalítica y conservación de herramientas de cobre únicas de la Edad oscura del Antiguo Egipto Resumen: El artículo presentó una investigación multianalítica de un conjunto único de herramientas de cobre que se remonta a la Edad Oscura, de la tumba de KHENNU Y APA-EM-SA-F (289) en el sur de Memphis, Saqqara. -
Contrasting Styles of Lead-Zinc-Barium Mineralization in the Lower Benue Trough, Southeastern Nigeria
EARTH SCIENCES RESEARCH JOURNAL Earth Sci. Res. J. Vol. 21, No. 1 (March, 2017): 7 - 16 ORE DEPOSITS Contrasting styles of lead-zinc-barium mineralization in the Lower Benue Trough, Southeastern Nigeria Ifeanyi Andrew Oha*,1, Kalu Mosto Onuoha1, Silas Sunday Dada2 1Department of Geology, University of Nigeria, Nsukka. 2Kwara State University Malete *[email protected] ABSTRACT Keywords: Lead-Zinc-Barium mineralization, In the Lower Benue Trough of Southeastern Nigeria, lead-zinc-barium mineralization occurs as widely distributed Benue Trough, epigenetic, vein deposits. epigenetic fracture-controlled vein deposits which are restricted to Albian – Turonian sediments. Detailed field studies carried out in Ishiagu, Enyigba-Ameki-Ameri, Wanikande-Wanakom, and Gabu-Oshina which together constitute the four main areas of mineralization in the Lower Benue Trough, show that mineralization appears restricted to NW-SE and N-S fractures while the more common NE-SW fractures are barren. Apart from the Enyigba area, igneous bodies are found in the vicinity of the ore deposits while in the Wanikande area, barite veins and veinlets were observed to be closely interwoven with intrusive bodies. The host lithologies are highly varied, ranging from shales to siltstones, sandstones and occasionally igneous bodies. The ore assemblage also varies remarkably, with lead:zinc:barium ratios ranging from approximately 3:1:0 at Ishiagu, to 2:1:0 at Enyigba, 1:0:2 at Wanikande and nearly 100% barite at Gabu-Oshina. Thus, there is a remarkable increase in barite content from the southwest (Ishiagu) to the northeast (Gabu). The characteristics of the ore deposits roughly fit the base metal type mineralization known as clastic dominated lead-zinc-barium deposits. -
Wickenburgite Pb3caal2si10o27² 3H2O
Wickenburgite Pb3CaAl2Si10O27 ² 3H2O c 2001 Mineral Data Publishing, version 1.2 ° Crystal Data: Hexagonal. Point Group: 6=m 2=m 2=m: Tabular holohedral crystals, dominated by 0001 and 1011 , to 1.5 mm. As spongy aggregates of small, highly perfect f g f g individuals; as subparallel aggregates or rosettes; granular. Physical Properties: Cleavage: 0001 , indistinct. Tenacity: Brittle but tough. Hardness = 5 D(meas.) = 3.85 D(cfalc.) g= 3.88 Fluoresces dull orange under SW UV. Optical Properties: Transparent to translucent. Color: Colorless to white; rarely salmon-pink. Luster: Vitreous. Optical Class: Uniaxial ({). Dispersion: r < v; moderate. ! = 1.692 ² = 1.648 Cell Data: Space Group: P 63=mmc: a = 8.53 c = 20.16 Z = 2 X-ray Powder Pattern: Near Wickenburg, Arizona, USA. 10.1 (100), 3.26 (80), 3.93 (60), 3.36 (40), 2.639 (40), 5.96 (30), 5.04 (30) Chemistry: (1) (2) SiO2 42.1 40.53 Al2O3 7.6 6.88 PbO 44.0 45.17 CaO 3.80 3.78 H2O 3.77 3.64 Total 101.27 100.00 (1) Near Wickenburg, Arizona, USA. (2) Pb3CaAl2Si10O24(OH)6: [needsnew??formula] Occurrence: In oxidized hydrothermal veins, carrying galena and sphalerite, in quartz and °uorite gangue (near Wickenburg, Arizona, USA). Association: Phoenicochroite, mimetite, cerussite, willemite, crocoite, duftite, hemihedrite, alamosite, melanotekite, luddenite, ajoite, shattuckite, vauquelinite, descloizite, laumontite. Distribution: In the USA, in Arizona, at several localities south of Wickenburg, Maricopa Co., including the Potter-Cramer property, Belmont Mountains, and the Moon Anchor mine; on dumps at a Pb-Ag-Cu prospect in the Artillery Peaks area, Mohave Co.; and in the Dives (Padre Kino) mine, Silver district, La Paz Co. -
About Our Mineral World
About Our Mineral World Compiled from series of Articles titled "TRIVIAL PURSUITS" from News Nuggets by Paul F. Hlava "The study of the natural sciences ought to expand the mind and enlarge the ability to grasp intellectual problems." Source?? "Mineral collecting can lead the interested and inquisitive person into the broader fields of geology and chemistry. This progression should be the proper outcome. Collecting for its own sake adds nothing to a person's understanding of the world about him. Learning to recognize minerals is only a beginning. The real satisfaction in mineralogy is in gaining knowledge of the ways in which minerals are formed in the earth, of the chemistry of the minerals and of the ways atoms are packed together to form crystals. Only by grouping minerals into definite categories is is possible to study, describe, and discuss them in a systematic and intelligent manner." Rock and Minerals, 1869, p. 260. Table of Contents: AGATE, JASPER, CHERT AND .............................................................................................................................2 GARNETS..................................................................................................................................................................2 GOLD.........................................................................................................................................................................3 "The Mystery of the Magnetic Dinosaur Bones" .......................................................................................................4 -
L<Ii. Tn / CORROSION, COLORANTS, CONSERVATION the Getty
>!"li H-t> /i f-f' i'*J"i T-t i"v /S 'f -».-K' Lin-it unj»L<ii. tn / CORROSION, COLORANTS, CONSERVATION DAVID A. SCOTT The Getty Conservation Institute Los Angeles Contents xi Foreword Timothy P. Whalen xii Preface l Introduction CHAPTER 1 CORROSION AND ENVIRONMENT 11 The Anatomy of Corrosion The electrochemical series 16 Some Historical Aspects of Copper and Corrosion Primitive wet-cell batteries? Early technologies with copper and iron Early history of electrochemical plating Copper in early photography Dezincification 32 Pourbaix Diagrams and Environmental Effects The burial environment The outdoor environment The indoor museum environment The marine environment 72 Copper in Contact with Organic Materials Positive replacement and mineralization of organic materials 77 The Metallography of Corroded Copper Objects 79 Corrosion Products and Pigments CHAPTER 2 OXIDES AND HYDROXIDES 82 Cuprite Properties of cuprite Natural cuprite patinas Intentional cuprite patinas Copper colorants in glasses and glazes 95 Tenorite Tenorite formation 98 Spertiniite 98 Conservation Issues CHAPTER 3 CHAPTER 5 BASIC COPPER CARBONATES BASIC SULFATES 102 Malachite 146 Historical References to Copper Sulfates Decorative uses of malachite 147 The Basic Copper Sulfates Malachite as a copper ore Brochantite and antlerite Nomenclature confusion Posnjakite Mineral properties Other basic sulfates Malachite as a pigment Malachite in bronze patinas 154 Environment and Corrosion Isotope ratios to determine corrosion Atmospheric sulfur dioxide environment Microenvironment