Nonenzymatic RNA Oligomerization at the Mineral–Water Interface
Total Page:16
File Type:pdf, Size:1020Kb
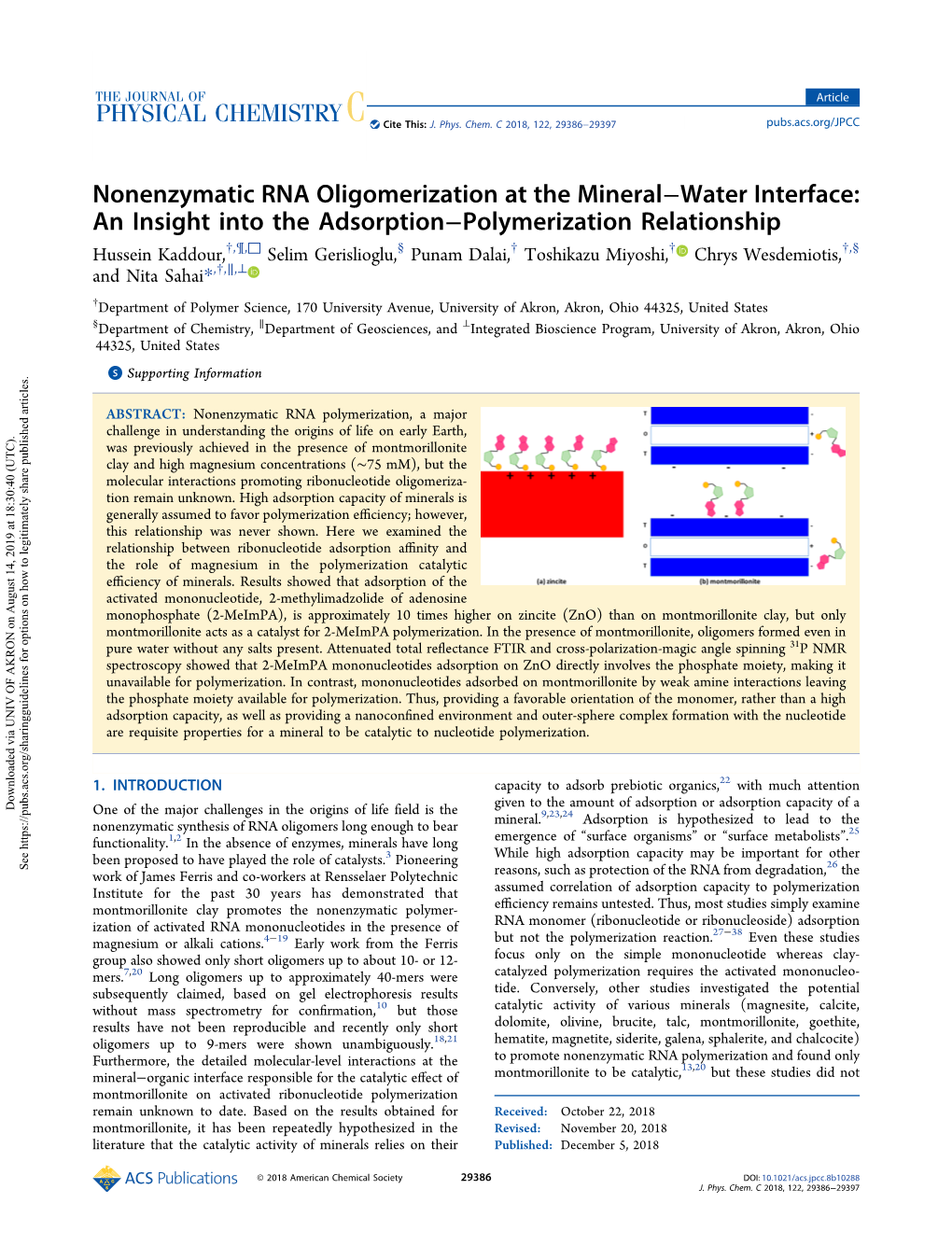
Load more
Recommended publications
-
United States Patent Office Patented Oct
3,346,562 United States Patent Office Patented Oct. 10, 1967 2 3,346,562 cg METHOD FOR THE PRODUCTION OF PO-CE Base RBONUCLEOSDE-5'-PHOSPHATE / O Mikio Honjo, Takatsuki, and Ryuji Maremoto, Minoo, Cl EO Japan, assignors to Takeda Chemical industries, Ltd., Osaka, Japan No Drawing. Filed May 31, 1966, Ser. No. 553,718 Claims priority, application Japan, May 29, 1965, R. X R. 40/31,814 9 Claims. (Cl. 260-21.5) HO. O. BIO O 10 N1 N1 This invention is concerned with a method for the pro Po-H, Base Po-H, Base duction of ribonucleoside-5'-phosphate. EIO k" wE+ EO k". Ribonucleoside-5'-phosphate is very useful as condi H HO - ment for food and also in the pharmaceutical industry, O O OH OH and has been chemically produced by at first protecting 15 X the hydroxyl groups at the 2'- and 3'-positions of its ribose R1 R2 moiety with acyl or isopropylidene groups and then phos phorylating the 5'-hydroxyl group of the thus-protected RN compound with pentavalent phosphorus compound such C=O: aliphatic ketone or aromatic aldehyde as phosphorus pentachloride, phosphorus oxychloride, 20 R?2 etc., followed by removing the protecting groups. As "ribonucleoside' in the present method there are However, this hitherto-known method requires a long used those containing purine base such as adenosine, time (about 7 to about 30 hours) for completing the pro inosine, etc. or those containing pyrimidine base such as tection and phosphorylation, and therefore is not desirable uridine, cytidine, etc. As the aliphatic ketone having 3 from an industrial viewpoint. -
SFU Thesis Template Files
Towards a Prebiotically Plausible Mechanism for the Emergence of RNA, Ribozymes and the RNA World by Lyssa Lynn Martin B.Sc., Thompson Rivers University, 2011 Thesis Submitted in Partial Fulfillment of the Requirements for the Degree of Master of Science in the Department of Molecular Biology and Biochemistry Faculty of Science Lyssa Lynn Martin 2015 SIMON FRASER UNIVERSITY Summer 2015 Approval Name: Lyssa Lynn Martin Degree: Master of Science Title: Towards a Prebiotically Plausible Mechanism for the Emergence of RNA, Ribozymes and the RNA World Examining Committee: Chair: Dr. Fredric Pio Associate Professor Peter Unrau Senior Supervisor Professor Dipankar Sen Supervisor Professor Niles Lehman Supervisor Professor Portland State University Department of Chemistry Nancy Forde Internal Examiner Associate Professor Department of Physics Date Defended/Approved: August 25, 2015 ii Abstract Available evidence, both in vitro and in vivo, attests to the descent of life from the RNA World; however, the prebiotic genesis of such RNA life remains ambiguous. How did The RNA World emerge from the abiotic chemistry on the Archean Earth? Montmorillonite clays have been shown to catalyze the polymerization of activated nucleotides (eg. adenosine 5′ phosphorimidazolide) into RNA, but polymerization has not previously been demonstrated for a prebiotically plausible nucleotide such as cyclic 2′, 3′-adenosine monophosphate (A>p). I reacted A>p in the presence of montmorillonite clay and could detect RNA polymers up to 5 nucleotides in length using a combination of HPLC, enzymatic labeling and mass spectrometry. This chemistry was found to be sensitive to pH and temperature. Reactions at pH 6 were found to produce more polymerization products than reactions at pH 7 or 8. -
Effects of Allopurinol and Oxipurinol on Purine Synthesis in Cultured Human Cells
Effects of allopurinol and oxipurinol on purine synthesis in cultured human cells William N. Kelley, James B. Wyngaarden J Clin Invest. 1970;49(3):602-609. https://doi.org/10.1172/JCI106271. Research Article In the present study we have examined the effects of allopurinol and oxipurinol on thed e novo synthesis of purines in cultured human fibroblasts. Allopurinol inhibits de novo purine synthesis in the absence of xanthine oxidase. Inhibition at lower concentrations of the drug requires the presence of hypoxanthine-guanine phosphoribosyltransferase as it does in vivo. Although this suggests that the inhibitory effect of allopurinol at least at the lower concentrations tested is a consequence of its conversion to the ribonucleotide form in human cells, the nucleotide derivative could not be demonstrated. Several possible indirect consequences of such a conversion were also sought. There was no evidence that allopurinol was further utilized in the synthesis of nucleic acids in these cultured human cells and no effect of either allopurinol or oxipurinol on the long-term survival of human cells in vitro could be demonstrated. At higher concentrations, both allopurinol and oxipurinol inhibit the early steps ofd e novo purine synthesis in the absence of either xanthine oxidase or hypoxanthine-guanine phosphoribosyltransferase. This indicates that at higher drug concentrations, inhibition is occurring by some mechanism other than those previously postulated. Find the latest version: https://jci.me/106271/pdf Effects of Allopurinol and Oxipurinol on Purine Synthesis in Cultured Human Cells WILLIAM N. KELLEY and JAMES B. WYNGAARDEN From the Division of Metabolic and Genetic Diseases, Departments of Medicine and Biochemistry, Duke University Medical Center, Durham, North Carolina 27706 A B S TR A C T In the present study we have examined the de novo synthesis of purines in many patients. -
The Origin of Life in the Solar System: Current Issues
Annual Reviews www.annualreviews.org/aronline Annu.Rev. EarthPlanet. Sci. 1995.23:215-49 Copyright~) 1995by AnnualReviews Inc. All rights reserved THE ORIGINOF LIFE IN THE SOLAR SYSTEM:Current Issues Christopher E Chyba White HouseFellow, National Security Council~Old Executive Office Building, Washington DC20506 Gene D. McDonald 2 Scripps Institution of Oceanography,University of California, San Diego, La Jolla, California 92093 I can see no other escapefrom this dilemma(lest our true aim be lost for ever) than that some of us shouldventure to embarkon a synthesis of facts and theories, albeit with second-hand and incompleteknowledge of someof them--and at the risk of makingfools of ourselves. Erwin Schr0dinger, What is Life? INTRODUCTION Progress in the study of the origins of life was dramatic during the decade of the 1980s. Primordial Earth was put morecompletely into the context of early Solar Systemhistory, and as this planetary view provided newinsights, other by Stanford University Libraries on 04/23/05. For personal use only. Solar Systemenvironments took on greater interest as points of comparison. Workon the energy sources needed to synthesize organic monomersor more sophisticated products on prebiotic Earth expandedwidely, with a numberof provocativenew ideas vying for attention. But mostimportantly, the elucidation Annu. Rev. Earth. Planet. Sci. 1995.23:215-249. Downloaded from arjournals.annualreviews.org of a putative RNAworld held out the hope for resolution of long-standing difficulties in the origin of DNA-proteinorganisms. This reviewis intended to report on someof the current issues in origins-of- life and exobiology research. Wetherefore cannot hope to present a compre- hensive history of the subject. -
Competitive Inhibition of Beef Heart Cyclic AMP Phosphodiesterase by Cytokinins and Related Compounds (Cyclic AMP Metabolism/Intracellular Cyclic AMP Concentration)
Proc. Nat. Acad. Sci. USA Vol. 71, No. 12, pp. 4670-4674, December 1974 Competitive Inhibition of Beef Heart Cyclic AMP Phosphodiesterase by Cytokinins and Related Compounds (cyclic AMP metabolism/intracellular cyclic AMP concentration) SIDNEY M. HECHT*, ROBERT D. FAULKNER, AND S. D. HAWRELAK Department of Chemistry, Massachusetts Institute of Technology, Cambridge, Mass. 02139 Communicated by Nelson J. Leonard, September 9, 1974 ABSTRACT Two cytokinins and four related analogs, also shown to contain detectable adenylate cyclase activity, none of which is a cyclic ribonucleotide, have been shown suggesting that the cytokinins might function by raising the to act as competitive inhibitors of the high Km cyclic-AMP phosphodiesterase (3': 5'-cyclic-AMP 5'-nucleotidohydro- intracellular level of cyclic AMP (18). If operative in mouse lase, EC 3.1.4.1-7) activity from beef heart. Weak inhibition fibroblasts this phenomenon might also explain the observed of the low Km cyclic AMP phosphodiesterase activity was growth inhibition of such cells by cytokinins (S. M. Hecht and also observed, suggesting a possible mechanism for regula- R.. B. Frye, in preparation), since it has been shown that there tion of intracellular cyclic AMP levels by the exogenously added compounds. In addition to the kinetic data, ob- is an inverse relationship between intracellular cyclic AMP tained on the six inhibitors in four different heterocyclic concentration and growth in fibroblasts (19). series, 15 other cytokinins and related compounds have To further explore the apparent involvement of exogenously been shown to inhibit the high Km cyclic AMP phospho- added cytokinins in cyclic AMP metabolism, we have in- diesterase activity at single concentrations of substrate vestigated the kinetics of interaction of certain cytokinins, and and inhibitor. -
Download Product Insert (PDF)
PRODUCT INFORMATION Guanosine Item No. 27702 CAS Registry No.: 118-00-3 Synonyms: Guanine Ribonucleoside, NSC 19994 N O MF: C10H13N5O5 FW: 283.2 N O OH Purity: ≥98% N N UV/Vis.: λmax: 254 nm Supplied as: A crystalline solid H OH OH H N Storage: -20°C 2 Stability: ≥2 years Information represents the product specifications. Batch specific analytical results are provided on each certificate of analysis. Laboratory Procedures Guanosine is supplied as a crystalline solid. A stock solution may be made by dissolving the guanosine in the solvent of choice, which should be purged with an inert gas. Guanosine is soluble in the organic solvent DMSO at a concentration of approximately 30 mg/ml. Guanosine is sparingly soluble in aqueous buffers. For maximum solubility in aqueous buffers, guanosine should first be dissolved in DMSO and then diluted with the aqueous buffer of choice. Guanosine has a solubility of approximately 0.16 mg/ml in a 1:5 solution of DMSO:PBS (pH 7.2) using this method. We do not recommend storing the aqueous solution for more than one day. Description Guanosine is a purine nucleoside that is comprised of the purine base guanine attached to a ribose moiety.1 Mono-, di-, tri-, and cyclic monophosphorylated forms of guanosine (GMP, GDP, GTP, and cGMP, respectively) are essential for a variety of endogenous biochemical processes, such as signal transduction, metabolism, and RNA synthesis.2-4 References 1. Voet, D. and Voet, J.G. 3rd ed., John Wiley & Sons, Hoboken, NJ (2004). 2. Hanson, R.W. and Garber, A.J. -
RNA Transcription (UV-Induced Crosslinkdng/Two-Dimensional Gel Electrophoresis) ISMO ULMANEN, BARBARA A
Proc. Nati Acad. Sci. USA Vol. 78, No. 12, pp. 7355-7359, December 1981 Biochemistry Role of two of the influenza virus core P proteins in recognizing cap 1 structures (m7GpppNm) on RNAs and in initiating viral RNA transcription (UV-induced crosslinkdng/two-dimensional gel electrophoresis) ISMO ULMANEN, BARBARA A. BRONI, AND ROBERT M. KRUG Molecular Biology and Genetics Unit of the Graduate School, Memorial Sloan-Kettering Cancer Center, New York, New York 10021 Communicated by Aaron J. Shatkin, August 21, 1981 ABSTRACT Purified influenza viral cores catalyze the entire triphosphate (6). This guanosine incorporation is apparently process of viral RNA transcription, which includes the endo- directed by the penultimate cytosine residue at the 3' end of nucleolytic cleavage of heterologous RNAs containing cap 1 the eight virion RNA (vRNA) templates (6). In the presence of (m7GpppNm) structures to generate capped primers 10-13 nu- all four triphosphates, the viral RNA transcripts are then cleotides long, the initiation oftranscription via the incorporation elongated. ofa guanosine residue onto the primers, and elongation ofthe viral This entire reaction is catalyzed by purified viral cores (nu- mRNAs [Plotch, S. J., Bouloy, M., Ulmanen, I. & Krug, R. M. cleocapsids) (6), which contain four known virus-specific pro- (1980) Cell 23, 847-858]. To identify which viral core protein (nu- teins: the nucleocapsid protein (NP), which constitutes the cleocapsid protein, P1, P2, or P3) recognizes the cap 1 structure majority (about 92%) of the protein, and the three P proteins on the RNA primer, we irradiated (UV) endonuclease reactions (6, 7). Studies with temperature-sensitive virus mutants indi- carried out by viral cores in the absence of ribonucleoside tri- that at least two of these P proteins are required for tran- phosphates, with a primer RNA labeled in its cap 1 structure with cate mo- scription (8, 9). -
Chemoenzymatic Synthesis of Nucleoside Analogs As Potential Medicinal Agents
Chemoenzymatic synthesis of nucleoside analogs as potential medicinal agents vorgelegt von M. Sc. Heba Yehia Mohamed ORCID: 0000-0002-3238-0939 von der Fakultät III-Prozesswissenschaften der Technischen Universität Berlin zur Erlangung des akademischen Grades Doktorin der Naturwissenschaften - Dr. rer. nat. – genehmigte Dissertation Promotionsausschuss: Vorsitzender: Prof. Dr. Roland Lauster, Medical Biotechnology, TU Berlin, Berlin Gutachter: Prof. Dr. Peter Neubauer, Bioprocess Engineering, TU Berlin, Berlin Gutachter: Prof. Dr. Jens Kurreck, Applied Biochemistry, TU Berlin, Berlin Gutachter: Prof. Dr. Vlada B. Urlacher, Biochemistry, Heinrich-Heine-Universität Düsseldorf, Düsseldorf Tag der wissenschaftlichen Aussprache: 16. Juli 2019 Berlin, 2019 Heba Y. Mohamed Synthesis of nucleoside analogs as potential medicinal agents Abstract Modified nucleosides are important drugs used to treat cancer, viral or bacterial infections. They also serve as precursors for the synthesis of modified oligonucleotides (antisense oligonucleotides (ASOs) or short interfering RNAs (siRNAs)), a novel and effective class of therapeutics. While the chemical synthesis of nucleoside analogs is challenging due to multi-step procedures and low selectivity, enzymatic synthesis offers an environmentally friendly alternative. However, current challenges for the enzymatic synthesis of nucleoside analogs are the availability of suitable enzymes or the high costs of enzymes production. To address these challenges, this work focuses on the application of thermostable purine and pyrimidine nucleoside phosphorylases for the chemo-enzymatic synthesis of nucleoside analogs. These enzymes catalyze the reversible phosphorolysis of nucleosides into the corresponding nucleobase and pentofuranose-1-phosphate and have already been successfully used for the synthesis of modified nucleosides in small scale. So far, the production of sugar-modified nucleosides has been a major challenge. -
Current Drugs to Treat Infections with Herpes Simplex Viruses-1 and -2
viruses Review Current Drugs to Treat Infections with Herpes Simplex Viruses-1 and -2 Lauren A. Sadowski 1,†, Rista Upadhyay 1,2,†, Zachary W. Greeley 1,‡ and Barry J. Margulies 1,3,* 1 Towson University Herpes Virus Lab, Department of Biological Sciences, Towson University, Towson, MD 21252, USA; [email protected] (L.A.S.); [email protected] (R.U.); [email protected] (Z.W.G.) 2 Towson University Department of Chemistry, Towson, MD 21252, USA 3 Molecular Biology, Biochemistry, and Bioinformatics Program, Towson University, Towson, MD 21252, USA * Correspondence: [email protected] † Authors contributed equally to this manuscript. ‡ Current address: Becton-Dickinson, Sparks, MD 21152, USA. Abstract: Herpes simplex viruses-1 and -2 (HSV-1 and -2) are two of the three human alphaher- pesviruses that cause infections worldwide. Since both viruses can be acquired in the absence of visible signs and symptoms, yet still result in lifelong infection, it is imperative that we provide interventions to keep them at bay, especially in immunocompromised patients. While numerous experimental vaccines are under consideration, current intervention consists solely of antiviral chemotherapeutic agents. This review explores all of the clinically approved drugs used to prevent the worst sequelae of recurrent outbreaks by these viruses. Keywords: acyclovir; ganciclovir; cidofovir; vidarabine; foscarnet; amenamevir; docosanol; nelfi- navir; HSV-1; HSV-2 Citation: Sadowski, L.A.; Upadhyay, R.; Greeley, Z.W.; Margulies, B.J. Current Drugs to Treat Infections 1. Introduction with Herpes Simplex Viruses-1 and -2. The world of anti-herpes simplex (anti-HSV) agents took flight in 1962 with the FDA Viruses 2021, 13, 1228. -
Ribonucleosides for an Artificially Expanded Genetic Information
Note pubs.acs.org/joc Ribonucleosides for an Artificially Expanded Genetic Information System † ‡ † ‡ † § † § Hyo-Joong Kim, , Nicole A. Leal, , Shuichi Hoshika, , and Steven A. Benner*, , † Foundation for Applied Molecular Evolution (FfAME), 720 SW Second Avenue, Suite 201, Gainesville, Florida 32601, United States ‡ Firebird Biomolecular Sciences LLC, 13709 Progress Boulevard, Box 17, Alachua, Florida 32615, United States § The Westheimer Institute for Science and Technology (TWIST), 720 SW Second Avenue, Suite 208, Gainesville, Florida 32601, United States *S Supporting Information ABSTRACT: Rearranging hydrogen bonding groups adds nucleobases to an artificially expanded genetic information system (AEGIS), pairing orthogonally to standard nucleotides. We report here a large-scale synthesis of the AEGIS nucleotide carrying 2- amino-3-nitropyridin-6-one (trivially Z) via Heck coupling and a hydroboration/oxidation sequence. RiboZ is more stable against epimerization than its 2′-deoxyribo analogue. Further, T7 RNA polymerase incorporates ZTP opposite its Watson−Crick comple- ment, imidazo[1,2-a]-1,3,5-triazin-4(8H)one (trivially P), laying grounds for using this “second-generation” AEGIS Z:P pair to add amino acids encoded by mRNA. ne of many accomplishments of synthetic biology over Because of their orthogonality, “first-generation” AEGIS pairs O the past two decades has been the generation of DNA are today used widely. In the clinic, AEGIS DNA is used to “ ” monitor the load of viruses in the blood of patients infected systems that have -
Overview of the Synthesis of Nucleoside Phosphates and Polyphosphates 13.1.6
Overview of the Synthesis of Nucleoside UNIT 13.1 Phosphates and Polyphosphates Phosphorylated nucleosides play a domi- ity to the synthesis. Side reactions can occur, nant role in biochemistry. Primary metabolism, such as depurination of the nucleoside, phos- DNA replication and repair, RNA synthesis, phorylation of the nucleobase, as well as chemi- protein synthesis, signal transduction, polysac- cal alteration of nucleobase analogs. Due to charide biosynthesis, and enzyme regulation their intrinsic reactivity, the synthesis of phos- are just a handful of processes involving these phoanhydride bonds is also synthetically chal- molecules. Literally thousands of enzymes use lenging. Phosphate anhydrides are phosphory- these compounds as substrates and/or regula- lating reagents that are readily degraded under tors. The need to obtain such compounds in acidic conditions. Finally, purification of syn- both labeled and unlabeled forms, as well as a thetic nucleotides can be problematic. Ionic burgeoning need for analogs, has driven the reagents, starting materials, and mixtures of development of a myriad of chemical and en- regioisomers (2′-, 3′-, 5′-phosphates) can be zymatic synthetic approaches. As chemical en- particularly difficult to separate from the de- tities, few molecules possess the wide array of sired product. densely packed functionality present in phos- In spite of the many potential difficulties phorylated nucleosides. This poses a formida- associated with nucleoside phosphorylation ble challenge to the synthetic chemist, one that and polyphosphorylation, a certain amount of has not yet been fully overcome. This overview success has been achieved in these areas. Given will address some common methods (synthetic the wealth of phosphorylating reagents avail- and enzymatic) used to construct phosphory- able, simple phosphorylation of nucleosides at lated nucleosides. -
Jump-Starting a Cellular World: Investigating the Origin of Life, from Soup to Networks Richard Robinson
Open access, freely available online Feature Jump-Starting a Cellular World: Investigating the Origin of Life, from Soup to Networks Richard Robinson physicist, a chemist, and a mathematician are stranded on a desert isle, when a can of food washes up on the A beach. The three starving scientists suggest, in turn, how to open the can and ease their hunger. The physicist suggests they hurl it upon the rocks to split it open, but this fails. The chemist proposes they soak it in the sea and let the salt water eat away at the metal; again, no luck. They turn in desperation to the mathematician, who begins, “Assume we have a can opener….” When discussing the evolution of life, biologists can often sound a bit like that mathematician. Beginning with a single cell, Darwinian evolution provides a simple, robust, and powerful algorithm for deriving all the astonishing richness of life, from bacteria to brains. Natural selection and other evolutionary forces, acting on surplus populations of replicating cells and multicellular organisms, lead inevitably DOI: 10.1371/journal.pbio.0030396.g001 to evolution and adaptation. Give biologists a cell, and they’ll Figure 1. Tynagh Chimneys give you the world. But beyond assuming the fi rst cell must A view from above a chimney fi eld, showing the chimneys (round black have somehow come into existence, how do biologists explain circles) and bubbles, which contain chambers. The object placed for its emergence from the prebiotic world four billion years ago? scale is two centimeters across. These fossil chimneys were formed well after life’s origin, but may be similar to those in which, according to one The short answer is that they can’t, yet.