A Multi-Wavelength Study of Protoplanetary Disks with Astronomical Unit Resolution Narges Jamialahmadi
Total Page:16
File Type:pdf, Size:1020Kb
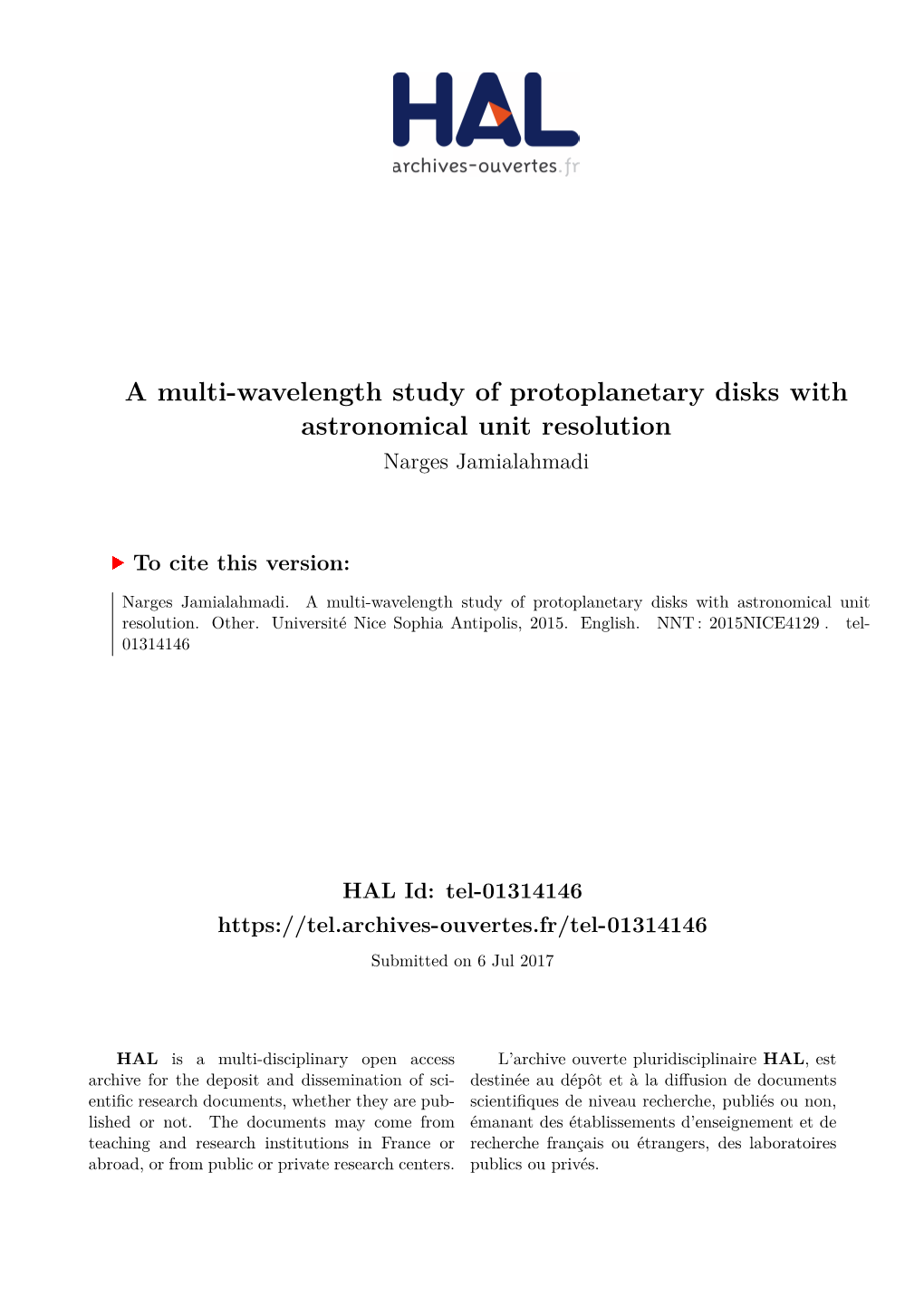
Load more
Recommended publications
-
The Astronomy of the Kamilaroi and Euahlayi Peoples and Their Neighbours
The Astronomy of the Kamilaroi and Euahlayi Peoples and Their Neighbours By Robert Stevens Fuller A thesis submitted to the Faculty of Arts at Macquarie University for the degree of Master of Philosophy November 2014 © Robert Stevens Fuller i I certify that the work in this thesis entitled “The Astronomy of the Kamilaroi and Euahlayi Peoples and Their Neighbours” has not been previously submitted for a degree nor has it been submitted as part of requirements for a degree to any other university or institution other than Macquarie University. I also certify that the thesis is an original piece of research and it has been written by me. Any help and assistance that I have received in my research work and the preparation of the thesis itself has been appropriately acknowledged. In addition, I certify that all information sources and literature used are indicated in the thesis. The research presented in this thesis was approved by Macquarie University Ethics Review Committee reference number 5201200462 on 27 June 2012. Robert S. Fuller (42916135) ii This page left intentionally blank Contents Contents .................................................................................................................................... iii Dedication ................................................................................................................................ vii Acknowledgements ................................................................................................................... ix Publications .............................................................................................................................. -
April 2019 BRAS Newsletter
Monthly Meeting April 8th at 7PM at HRPO (Monthly meetings are on 2nd Mondays, Highland Road Park Observatory). Speaker: Merrill Hess will speak on “The life cycle of stars." What's In This Issue? President’s Message Secretary's Summary Outreach Report Astrophotography Group Asteroid and Comet News Light Pollution Committee Report Globe at Night Recent BRAS Forum Entries Messages from the HRPO Science Academy Friday Night Lecture Series Special Presentation 13 April: “Skygazing—A Pursuer’s Guide” International Astronomy Day” American Radio Relay League Field Day Observing Notes – Cancer the Crab & Mythology Like this newsletter? See PAST ISSUES online back to 2009 Visit us on Facebook – Baton Rouge Astronomical Society Newsletter of the Baton Rouge Astronomical Society April 2019 © 2019 President’s Message As we move into spring hopefully the run of cloudy nights we had this winter will end. At the last meeting we finally did the drawing for the Meade ETX 90EC, which was won by Joel Tews. Congratulations and thanks to all who bought raffle tickets. I would like take this moment to congratulate Coy Wagoner on being published in the March 2019 Reflector. BRAS CRAWFISH BOIL There will be a crawfish boil on May 18, 2019 at the home of Michele and John. Club will provide the crawfish and trimmings, with side dishes by attendees. Put it on your calendar now, please. We will need a head count to know how many crawfish to buy. More details and a map will follow in next month’s newsletter. Raffle winner was Joel Tews VOLUNTEER AT HRPO: If any of the members wish to volunteer at HRPO, please speak to Chris Kersey, BRAS Liaison for BREC, to fill out the paperwork. -
Observational Diagnostics of Gas in Protoplanetary Disks
Noname manuscript No. (will be inserted by the editor) Observational diagnostics of gas in protoplanetary disks Andr´esCarmona October 2008. Review written for the proceedings of the conference "Origin and Evolution of Planets 2008", Ascona, Switzerland, 2008. Abstract Protoplanetary disks are composed primar- disk last?, how much material is available for forming ily of gas (99% of the mass). Nevertheless, relatively giant planets?, how do the density and the temperature few observational constraints exist for the gas in disks. of the disk vary as a function of the radius?, what are In this review, I discuss several observational diagnos- the dynamics of the disk?. tics in the UV, optical, near-IR, mid-IR, and (sub)-mm Although we have learned important insights from wavelengths that have been employed to study the gas disks from dust observations (see for example the re- in the disks of young stellar objects. I concentrate in views by Henning et al. 2006 and Natta et al. 2007), diagnostics that probe the inner 20 AU of the disk, the dust presents several limitations: (i) dust spectral fea- region where planets are expected to form. I discuss the tures are broad; consequently, dust emission does not potential and limitations of each gas tracer and present provide kinematical information; (ii) dust properties are prospects for future research. expected to change during the planet formation pro- Keywords solar system formation · protoplanetary cess; therefore, quantities such as the gas-to-dust ra- disks · observations · gas · spectroscopy tio (needed to derive the disk mass from dust contin- uum emission in the (sub)-mm) are expected to strongly vary with respect to the conditions of the Interstellar Medium (ISM). -
Mar 2019 OBSERVER
THE OBSERVER OF THE TWIN CITY AMATEUR ASTRONOMERS Volume 44, Number 3 March 2019 INSIDE THIS ISSUE: 1«Editor’s Choice: Image of the Month – M42 2«President’s Note 3«Calendar of Celestial Events – March 2019 3«New & Renewing Members/Dues Blues/E-Mail List 4«This Month’s Phases of the Moon 4«This Month’s Solar Phenomena 4«Minutes of the February 9th Annual Meeting 5«AstroBits – News from Around the TCAA 7«Images from the 2019 Annual Meeting & Banquet 8«24” Moved to Dome on Saturday, February 16th 9«Prairie Sky Observatory Today 10«March 2019 with Jeffrey L. Hunt 13«NCRAL 2019 is Coming! 14«Public Viewing Sessions Schedule for 2019 14«TCAA Active on Facebook 15«TCAA Calendar of Events for 2019 15«HowTimeFlies 15«Renewing Your TCAA Membership 18«TCAA Treasurer’s Report as of February 28, 2019 EDITOR’S CHOICE: IMAGE OF THE MONTH – M42 This month’s Editor’s Choice is an image of the Great Orion nebula taken by Carl Wenning at Sugar Grove Observatory on February 27, 2012. Carl was aided in the capture of this image by Bob Finnigan and post-processing was conducted by Lee Green. According to Carl, “There’s not much to be impressed about with the quality of this image. It’s pretty much run of the mill, with much better work having been done by other club members in recent years. What is worthy of note, however, is how the club has the capacity to take out-of-this-world images using some of the most advanced astrophotographic equipment available to amateur astronomers anywhere on the planet. -
VISION: a Six-Telescope Fiber-Fed Visible Light Beam Combiner For
VISION: A Six-Telescope Fiber-Fed Visible Light Beam Combiner for the Navy Precision Optical Interferometer Eugenio V. Garcia1,2, Matthew W. Muterspaugh3,4, Gerard van Belle1, John D. Monnier5, Keivan G. Stassun2, Askari Ghasempour6, James H. Clark7, R. T. Zavala8, James A. Benson8, Donald J. Hutter8, Henrique R. Schmitt7, Ellyn K. Baines7, Anders M. Jorgensen10, Susan G. Strosahl1, Jason Sanborn1, Stephen J. Zawicki1, Michael F. Sakosky1, Samuel Swihart9 Abstract Visible-light long baseline interferometry holds the promise of advancing a number of impor- tant applications in fundamental astronomy, including the direct measurement of the angular diameters and oblateness of stars, and the direct measurement of the orbits of binary and mul- tiple star systems. To advance, the field of visible-light interferometry requires development of instruments capable of combining light from 15 baselines (6 telescopes) simultaneously. The Vis- ible Imaging System for Interferometric Observations at NPOI (VISION) is a new visible light beam combiner for the Navy Precision Optical Interferometer (NPOI) that uses single-mode fibers to coherently combine light from up to six telescopes simultaneously with an image-plane combination scheme. It features a photometric camera for calibrations and spatial filtering from single-mode fibers with two Andor Ixon electron multiplying CCDs. This paper presents the VISION system, results of laboratory tests, and results of commissioning on-sky observations. A new set of corrections have been determined for the power spectrum and bispectrum by taking into account non-Gaussian statistics and read noise present in electron-multipying CCDs to en- able measurement of visibilities and closure phases in the VISION post-processing pipeline. -
THE STAR FORMATION NEWSLETTER an Electronic Publication Dedicated to Early Stellar Evolution and Molecular Clouds
THE STAR FORMATION NEWSLETTER An electronic publication dedicated to early stellar evolution and molecular clouds No. 191 — 9 Nov 2008 Editor: Bo Reipurth ([email protected]) Abstracts of recently accepted papers A Search for H2CO 6 cm Emission toward Young Stellar Objects. III. VLA Observations E. D. Araya1,2,3, P. Hofner2,3, W. M. Goss2, H. Linz4, S. Kurtz5, and L. Olmi6,7 1 Department of Physics and Astronomy, University of New Mexico, MSC07 4220, Albuquerque, NM 87131, USA. 2 National Radio Astronomy Observatory, P.O. Box 0, Socorro, NM 87801, USA. 3 Physics Department, New Mexico Institute of Mining and Technology, 801 Leroy Place, Socorro, NM 87801, USA. 4 Max-Planck-Institut f¨ur Astronomie, K¨onigstuhl 17, D-69117 Heidelberg, Germany. 5 Centro de Radioastronoma y Astrofsica, UNAM, Apdo. Postal 3-72, 58089, Morelia, Michoac´an, Mexico. 6 Physics Department, University of Puerto Rico at Rio Piedras, P.O. Box 23343, San Juan, PR 00931. 7 Istituto di Radioastronomia, INAF, Sezione di Firenze, Largo Enrico Fermi 5, I-50125 Florence, Italy. We report the results of our third survey for formaldehyde (H2CO) 6 cm maser emission in the Galaxy. Using the Very Large Array, we detected two new H2CO maser sources (G23.01-0.41 and G25.83-0.18), thus increasing the sample of known H2CO maser regions in the Galaxy to seven. We review the characteristics of the G23.01-0.41 and G25.83-0.18 star-forming regions. The H2CO masers in G23.01-0.41 and G25.83-0.18 share several properties with the other known H2CO masers, in particular, emission from rich maser environments and close proximity to very young massive stellar objects. -
Circumstellar Disk Astrochemistry Student: Johanna Teske American University Advisor: Dr
–1– Circumstellar Disk Astrochemistry Student: Johanna Teske American University Advisor: Dr. Nathan Harshman DTM Advisors: Dr. Alycia Weinberger & Dr. Aki Roberge Fall 2007—Spring 2008 Graduating with Honors in Physics Circumstellar Disk Astrochemistry Johanna Teske1 & Alycia Weinberger2 & Aki Roberge3 ABSTRACT One of the most exciting and promising fields in modern astronomy is the detection and classification of extrasolar planetary systems. The processes that produce other worlds begin early the in the life of the host star with formation of an equatorial disk of gas and dust to stabilize the rapidly contracting and rotating protostar. Planetary studies rely heavily on these circumstellar disks, which are found at various stages of stellar life and ultimately make up the diverse range of planets we see today. There are important stages of disk evolution that are not well understood; this Capstone focuses on debris disks, representing an intermediate stage between gas-rich protoplanetary disks and established planetary systems. To further understand the complex interaction of gas and dust in these disks, the optical spectra of A-shell stars were examined via measurement of absorption line strength and radial velocity to characterize their circumstellar material and diagnose why some stars show little evidence for a dust component, while others have significant infrared excess. 1. Introduction In modern astronomy, one of the most exciting fields attracting scientists from across disciplines is the detection and characterization of extrasolar planets, unique and exotic worlds that have been postulated to exist for centuries but only recently confirmed. The rate of discovery of “alien” worlds has accelerated tremendously within the last decade alone, thanks in great part to the enhanced technologies and observa- tional facilities, and shows no sign of slowing down. -
NCRAL Northern Lights Autumn 2019
• complete the proposal For the Astronomical Bucket List INSIDE THIS ISSUE OF Northern Lights observing program and re-submit it (noting Regional NCRAL Chair’s Message……………..................................……………1 membership support) to the Astronomical League for ALCon 2019 in Review……………………………………………………………..2 formal approval at ALCon 2020, and Secretary-Treasurer’s Report…………………………………………………..4 • seek 501(c)(3) non-profit status For NCRAL so that we can NCRAL 2020 Approaching……………………………………………………….5 provide tax write ofFs For those willing to contribute to the Total Solar Eclipse From La Higuera, Chile: July 02, 2019……………5 Region’s educational and service activities. November 11, 2019 Transit of Mercury……………………..…………….6 Venus Article Correction…….…………………………………….……………..8 Achieving each oF these goals will require considerable Venus Entering Evening Sky……………………………………………………9 time and eFFort, and I hope to work on one of these goals each Mars Until Retrograde 2020……………………………………………..……10 quarter. For now, I’m happy to announce that I earlier shared NCRAL Observing Program Approved – It’s Official………………...17 draft guidelines For the NCRAL Seasonal Messier Marathons NCRAL Seasonal Messier Marathon Observing Program…………17 with the Regional Council For review. Having hear nothing but First NCRAL Member Recruitment Mini Grant Moving Along….19 praise For the new program, I am publishing the guidelines for Add Your Email Address to the NCRAL Member Database……...20 program in this issue oF Northern Lights. The First of the three Updating the Regional Council Email Database……………………...20 goals For my 2019-2020 term has been accomplished. Call For 2020 NCRAL Nominations…………………………………….…..20 I’m happy to announce that our host For NCRAL 2019, Future NCRAL Regional Conventions……………………..…………..….22 Popular Astronomy Club, turned a proFit hosting the spring NCRAL & AL on Facebook…………………………..…………………………..23 meeting at Moline, Illinois. -
Contrat Quinquennal 2016‐2020
Contrat Quinquennal 2016‐2020 Annexe 6 du dossier d’évaluation : Production Scientifique 19/09/2014 AERES Vague A IPAG / UMR5274 ANNEXE 6 : IPAG 2016-2020 PRODUCTION SCIENTIFIQUE Publications ACL par année et par équipe 100 90 80 astromol 70 cristal 60 fost 50 40 planeto 30 sherpas 20 multi 10 0 2009 2010 2011 2012 2013 Publications ACL par équipe 2009‐2013 sherpas 17% astromol 21% planeto cristal 15% 8% fost 39% 07/07/2014 PublIPAG 1 15 Principaux support de publication 2009‐2013 250 Nature Journal of Geophysical Research (Planets) 200 The Astrophysical Journal Supplement Series Journal of Space Weather and Space Climate Astroparticle Physics The Astronomical Journal 150 Science Experimental Astronomy Geochimica et Cosmochimica Acta 100 Journal of Chemical Physics Planetary and Space Science Icarus 50 Monthly Notices of the Royal Astronomical Society The Astrophysical Journal Astronomy and Astrophysics 0 2009 2010 2011 2012 2013 07/07/2014 PublIPAG 2 Liste des publications 2009‐2014 IPAG publications are counted by team. The sum of all team papers (1122) is larger than the number of papers for the whole laboratory (1068) because some publications are written in common between 2 ou more teams. There are 54 (=1122‐1068) 'multi‐team' papers during the period. ACL refereed articles (1068) ........................................................................................................ 4 ACL astromol (242) ................................................................................................................................... -
Curriculum Vitae
Curriculum Vitae Dr. Theo ten Brummelaar August 8, 2018 Home Work XXXXXXXXXXXXXXXXX The CHARA Array of Altadena, CA 91001 Georgia State University U.S.A. PO Box 48 Mount Wilson, CA 91023, U.S.A. Ph: (XXX)-XXX-XXXX Ph: (+1 626)-796-8607 Cell: (XXX)-XXX-XXXX FAX: (+1 626)-796-6717 email: [email protected] email: [email protected] WWW: http://www.astro.gsu.edu/∼theo Date of Birth: Second of May, 1962. Citizenship: United States & Australia. Employment History: • Center for High Angular Resolution Astronomy (CHARA - Georgia State University): { September 2015 - Present: Director of the CHARA Array { March 2001 - July 2015: Associate Director of CHARA { May 1996 - February 2001 : Research Scientist II. { April 1993 - April 1996 : Postdoctoral Research Fellow. • University of Sydney: 2008-present Honorary Appointment at the School of Physics • Partner in CHIP software company: January 1989 - January 1993. Clients included: { University of New South Wales: Civil Engineering Department, Road illumina- tion simulation software. { University of Sydney: Music Department, MIDI Music/Studio control software. { Commonwealth Scientific and Industrial Research Organisation: Non-destructive test group, FTI a large gear testing instrument. • Lamplight Theatre: 1990. Actor. • Continuing Education Programme (Sydney University) : March 1989 - 1991. Lecturer in a basic Astronomy course for adults entitled `Introduction to Astronomy'. 1 • Special Broadcasting Service : April 1980 - December 1992. Part-time Radio Broadcast Operator, Producer and Sound Engineer. • Commonwealth Scientific and Industrial Research Organisation (CSIRO), Division of Applied Physics : Experimental Scientist December 1985 - November 1988. Working on the development of a new 3-D ultrasonic scanning and imaging system for the metal and airline industries. -
Introduction Who Was Sir William Herschel?
Astronomers’ Observing Guides Other Titles in This Series Star Clusters and How to Observe Them Mark Allison Saturn and How to Observe it Julius Benton Nebulae and How to Observe Them Steven Coe The Moon and How to Observe It Peter Grego Supernovae and How to Observe Them Martin Mobberley Double and Multiple Stars and How to Observe Them James Mullaney Galaxies and How to Observe Them Wolfgang Steinicke & Richard Jakiel Forthcoming Title in This Series Total Solar Eclipses and How to Observe Them Martin Mobberley James Mullaney, F.R.A.S. The Herschel Objects and How to Observe Them with 90 Illustrations James Mullaney Rehoboth Beach Delaware USA [email protected] Series Editor: Dr. Mike Inglis, BSc, MSc, Ph.D. Fellow of the Royal Astronomical Society Suffolk County Community College New York, USA [email protected] Library of Congress Control Number: 2007923721 ISBN-13: 978-0-387-68124-5 e-ISBN-13: 978-0-387-68125-2 Printed on acid-free paper. © 2007 Springer Science+Business Media, LLC All rights reserved. This work may not be translated or copied in whole or in part without the written permission of the publisher (Springer Science+Business Media, LLC, 233 Spring Street, New York, NY 10013, USA), except for brief excerpts in connection with reviews or scholarly analysis. Use in connection with any form of information storage and retrieval, electronic adaptation, computer software, or by similar or dissimilar methodology now known or hereafter developed is forbidden. The use in this publication of trade names, trademarks, service marks, and similar terms, even if they are not identified as such, is not to be taken as an expression of opinion as to whether or not they are subject to proprietary rights. -
CO EMISSION from the INNER DISK AROUND INTERMEDIATE-MASS STARS Marc George Berthoud, Ph.D
CO EMISSION FROM THE INNER DISK AROUND INTERMEDIATE-MASS STARS A Dissertation Presented to the Faculty of the Graduate School of Cornell University in Partial Fulfillment of the Requirements for the Degree of Doctor of Philosophy by Marc George Berthoud January 2008 c 2008 Marc George Berthoud ALL RIGHTS RESERVED CO EMISSION FROM THE INNER DISK AROUND INTERMEDIATE-MASS STARS Marc George Berthoud, Ph.D. Cornell University 2008 To investigate the interaction between young stars and their circumstellar ma- terial we conducted a spectral survey of Herbig Ae/Be stars in the K-band. Non- photospheric Brγ emission is observed in the spectra from most stars, indicating a similar Brγ flux as the cooler intermediate mass T-Tauri stars. We find that the emission is probably caused by winds or by hot disk gas. To study different methods to estimate accretion luminosity we compared our Brγ fluxes with other accretion indicators. The mismatch between the estimated accretion luminosities suggests that using Brγ could overestimate the Lacc by several orders of magnitude. We also observed CO overtone emission, but in a few objects only, perhaps because hot, dense gas is not common around these stars. We obtained high reso- lution spectra of the objects with observable CO overtone emission. These spectra allow us to establish constraints on the geometric distribution and physical char- acteristics of the emitting gas. We implemented a model of a hot gas disk to fit to our observations. Our results agree with previous conclusions, that 51 Oph is most likely a classical Be star, surrounded by a massive disk of hot gas.