Chandra X-Ray Spectroscopy of Focused Wind in the Cygnus X-1 System II
Total Page:16
File Type:pdf, Size:1020Kb
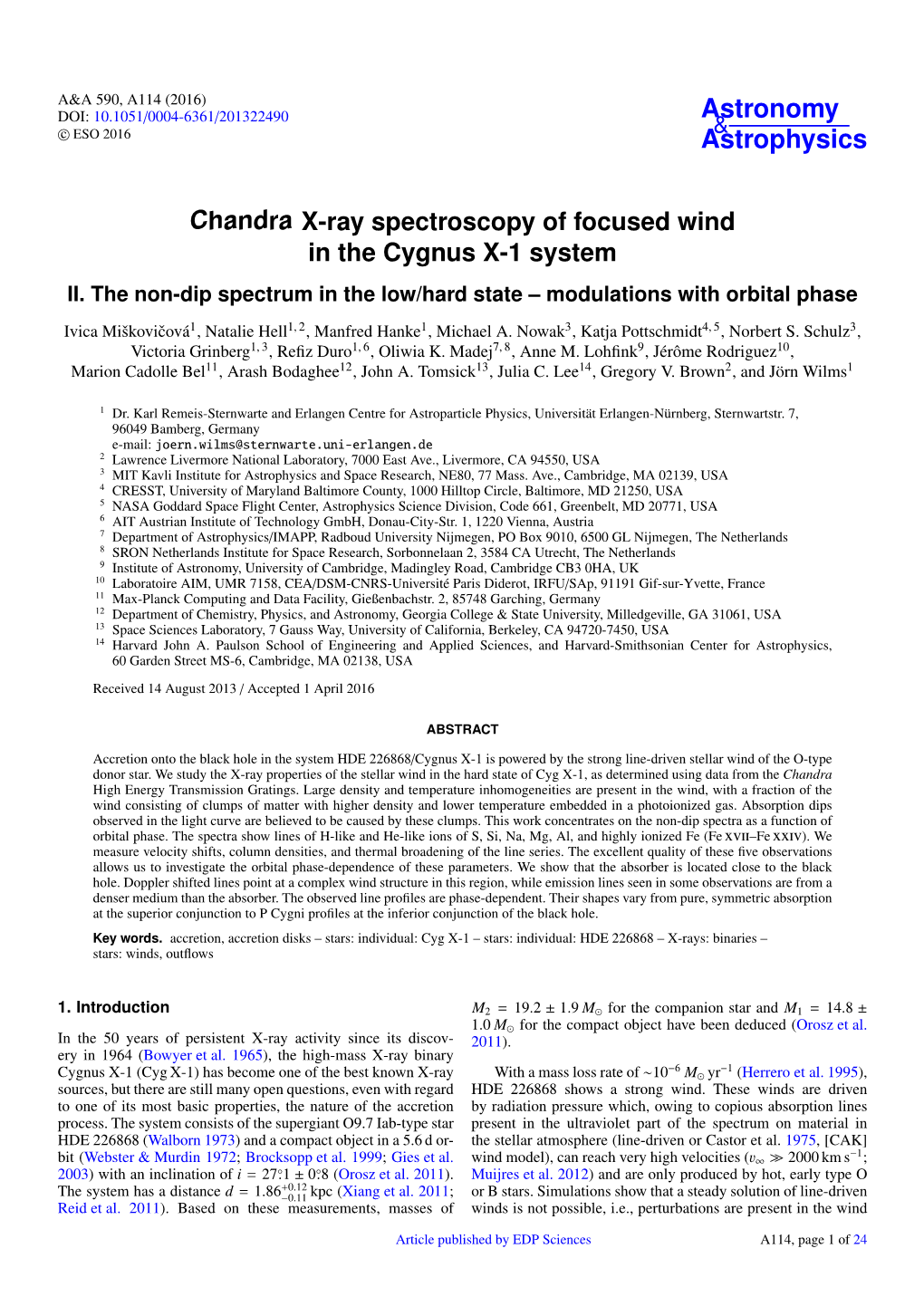
Load more
Recommended publications
-
Exploring Exoplanet Populations with NASA's Kepler Mission
SPECIAL FEATURE: PERSPECTIVE PERSPECTIVE SPECIAL FEATURE: Exploring exoplanet populations with NASA’s Kepler Mission Natalie M. Batalha1 National Aeronautics and Space Administration Ames Research Center, Moffett Field, 94035 CA Edited by Adam S. Burrows, Princeton University, Princeton, NJ, and accepted by the Editorial Board June 3, 2014 (received for review January 15, 2014) The Kepler Mission is exploring the diversity of planets and planetary systems. Its legacy will be a catalog of discoveries sufficient for computing planet occurrence rates as a function of size, orbital period, star type, and insolation flux.The mission has made significant progress toward achieving that goal. Over 3,500 transiting exoplanets have been identified from the analysis of the first 3 y of data, 100 planets of which are in the habitable zone. The catalog has a high reliability rate (85–90% averaged over the period/radius plane), which is improving as follow-up observations continue. Dynamical (e.g., velocimetry and transit timing) and statistical methods have confirmed and characterized hundreds of planets over a large range of sizes and compositions for both single- and multiple-star systems. Population studies suggest that planets abound in our galaxy and that small planets are particularly frequent. Here, I report on the progress Kepler has made measuring the prevalence of exoplanets orbiting within one astronomical unit of their host stars in support of the National Aeronautics and Space Admin- istration’s long-term goal of finding habitable environments beyond the solar system. planet detection | transit photometry Searching for evidence of life beyond Earth is the Sun would produce an 84-ppm signal Translating Kepler’s discovery catalog into one of the primary goals of science agencies lasting ∼13 h. -
Get Outside What to Look for in the Summer Sky Your Hosts of the Summer Sky Are Three Bright Stars — Vega, Altair and Deneb
Get Outside What to Look for in the Summer Sky Your hosts of the summer sky are three bright stars — Vega, Altair and Deneb. Together they make up the Summer Triangle. Look for the triangle in the east on a June evening, moving NORTH to overhead as the season progresses. Polaris The Big Dipper Deneb Cygnus Vega Lyra Hercules Arcturus EaST West Summer Triangle Altair Aquila Sagittarius Antares Turn the map so Scorpius the direction you are facing is at the Teapot the bottom. south facebook.com/KidsCanBooks @KidsCanPress GET OUTSIDE Text © 2013 Jane Drake & Ann Love Illustrations © 2013 Heather Collins www.kidscanpress.com Get Outside Vega The Keystone The brightest star in the Between Vega and Arcturus, Summer Triangle, Vega is look for four stars in a wedge or The summer bluish white. It is in the keystone shape. This is the body solstice constellation Lyra, the Harp. of Hercules, the Strongman. His feet are to the north and Every day from late Altair his arms to the south, making December to June, the The second-brightest star in his figure kneel Sun rises and sets a little the triangle, Altair is white. upside down farther north along the Altair is in the constellation in the sky. horizon. But about June Aquila, the Eagle. 21, the Sun seems to stop Keystone moving north. It rises in Deneb the northeast and sets in The dimmest star of the the northwest, seemingly Summer Triangle, Deneb would in the same spots for be the brightest if it were not so Hercules several days. -
Summer Constellations
Night Sky 101: Summer Constellations The Summer Triangle Photo Credit: Smoky Mountain Astronomical Society The Summer Triangle is made up of three bright stars—Altair, in the constellation Aquila (the eagle), Deneb in Cygnus (the swan), and Vega Lyra (the lyre, or harp). Also called “The Northern Cross” or “The Backbone of the Milky Way,” Cygnus is a horizontal cross of five bright stars. In very dark skies, Cygnus helps viewers find the Milky Way. Albireo, the last star in Cygnus’s tail, is actually made up of two stars (a binary star). The separate stars can be seen with a 30 power telescope. The Ring Nebula, part of the constellation Lyra, can also be seen with this magnification. In Japanese mythology, Vega, the celestial princess and goddess, fell in love Altair. Her father did not approve of Altair, since he was a mortal. They were forbidden from seeing each other. The two lovers were placed in the sky, where they were separated by the Celestial River, repre- sented by the Milky Way. According to the legend, once a year, a bridge of magpies form, rep- resented by Cygnus, to reunite the lovers. Photo credit: Unknown Scorpius Also called Scorpio, Scorpius is one of the 12 Zodiac constellations, which are used in reading horoscopes. Scorpius represents those born during October 23 to November 21. Scorpio is easy to spot in the summer sky. It is made up of a long string bright stars, which are visible in most lights, especially Antares, because of its distinctly red color. Antares is about 850 times bigger than our sun and is a red giant. -
Constellation at Your Fingertips: Cygnus
The National Optical Astronomy Observatory’s Dark Skies and Energy Education Program Constellation at Your Fingertips: Cygnus (Cygnus version adapted from Constellation at Your Fingertips: Orion at www.globeatnight.org) Grades: 3rd - 8th grade Overview: Constellation at Your Fingertips introduces the novice constellation hunter to a method for spotting the main stars in the constellation, Cygnus, the swan. Students will make an outline of the constellation used to locate the stars in Cygnus and visualize its shape. This lesson will engage children and first-time night sky viewers in observations of the night sky. The lesson links history, Greek mythology, literature, and astronomy. Once the constellation is identified, it may be easy to find the summer triangle. The simplicity of Cygnus makes locating and observing it exciting for young learners. More on Cygnus is at the Great World Wide Star Count at http://www.starcount.org. Materials for another citizen-science star hunt, Globe at Night, are available at http://www.globeatnight.org. Purpose: Students will use the this activity to visualize the constellation of Cygnus. This will help them more easily participate in the Great World Wide Star Count citizen-science campaign. The campaign asks participants to match one of 7 different charts of Cygnus with what the participant sees toward Cygnus. Chart 1 has only a few stars. Chart 7 has many. What they will be recording for the campaign is the chart with the faintest star they see. In many cases, observations over years will build knowledge of how light pollution changes over time. Why is this important to astronomers? What causes the variation year to year? The focus is on light pollution and the options we have as consumers when purchasing outdoor lighting and shields. -
The Kepler Characterization of the Variability Among A- and F-Type Stars I
A&A 534, A125 (2011) Astronomy DOI: 10.1051/0004-6361/201117368 & c ESO 2011 Astrophysics The Kepler characterization of the variability among A- and F-type stars I. General overview K. Uytterhoeven1,2,3,A.Moya4, A. Grigahcène5,J.A.Guzik6, J. Gutiérrez-Soto7,8,9, B. Smalley10, G. Handler11,12, L. A. Balona13,E.Niemczura14, L. Fox Machado15,S.Benatti16,17, E. Chapellier18, A. Tkachenko19, R. Szabó20, J. C. Suárez7,V.Ripepi21, J. Pascual7, P. Mathias22, S. Martín-Ruíz7,H.Lehmann23, J. Jackiewicz24,S.Hekker25,26, M. Gruberbauer27,11,R.A.García1, X. Dumusque5,28,D.Díaz-Fraile7,P.Bradley29, V. Antoci11,M.Roth2,B.Leroy8, S. J. Murphy30,P.DeCat31, J. Cuypers31, H. Kjeldsen32, J. Christensen-Dalsgaard32 ,M.Breger11,33, A. Pigulski14, L. L. Kiss20,34, M. Still35, S. E. Thompson36,andJ.VanCleve36 (Affiliations can be found after the references) Received 30 May 2011 / Accepted 29 June 2011 ABSTRACT Context. The Kepler spacecraft is providing time series of photometric data with micromagnitude precision for hundreds of A-F type stars. Aims. We present a first general characterization of the pulsational behaviour of A-F type stars as observed in the Kepler light curves of a sample of 750 candidate A-F type stars, and observationally investigate the relation between γ Doradus (γ Dor), δ Scuti (δ Sct), and hybrid stars. Methods. We compile a database of physical parameters for the sample stars from the literature and new ground-based observations. We analyse the Kepler light curve of each star and extract the pulsational frequencies using different frequency analysis methods. -
Scientists Discover Planetary System Orbiting Kepler-47 13 September 2012, by Dr
Scientists discover planetary system orbiting Kepler-47 13 September 2012, by Dr. Tony Phillips "The presence of a full-fledged planetary system orbiting Kepler-47 is an amazing discovery," says Greg Laughlin, professor of Astrophysics and Planetary Science at the University of California in Santa Cruz. "This is going to change the way we think about the formation of planets." The inner planet, Kepler-47b, closely circles the pair of stars, completing each orbit in less than 50 days. Astronomers think it is a sweltering world, where the destruction of methane in its super- heated atmosphere might lead to a thick global haze. Kepler-47b is about three times the size of Earth. The outer planet, Kepler-47c, orbits every 303 days. This puts it in the system's habitable zone, a This diagram compares our own solar system to band of orbits that are "just right" for liquid water to Kepler-47, a double-star system containing two planets, exist on the surface of a planet. But does this one orbiting in the so-called "habitable zone." This is the sweet spot in a planetary system where liquid water planet even have a surface? Possibly not. The might exist on the surface of a planet. Credit: NASA/JPL- astronomers think it is a gas giant slightly larger Caltech/T. Pyle than Neptune. The discovery of planets orbiting double stars means that planetary systems are even weirder News flash: The Milky Way galaxy just got a little and more abundant than previously thought. weirder. Back in 2011 astronomers were amazed when NASA's Kepler spacecraft discovered a "Many stars are part of multiple-star systems where planet orbiting a double star system. -
Far-Infrared Observations of a Massive Cluster Forming in the Monoceros R2 filament Hub? T
Astronomy & Astrophysics manuscript no. monr2_hobys˙final˙corrected c ESO 2017 December 5, 2017 Far-infrared observations of a massive cluster forming in the Monoceros R2 filament hub? T. S. M. Rayner1??, M. J. Griffin1, N. Schneider2; 3, F. Motte4; 5, V. K¨onyves5, P. Andr´e5, J. Di Francesco6, P. Didelon5, K. Pattle7, D. Ward-Thompson7, L. D. Anderson8, M. Benedettini9, J.-P. Bernard10, S. Bontemps3, D. Elia9, A. Fuente11, M. Hennemann5, T. Hill5; 12, J. Kirk7, K. Marsh1, A. Men'shchikov5, Q. Nguyen Luong13; 14, N. Peretto1, S. Pezzuto9, A. Rivera-Ingraham15, A. Roy5, K. Rygl16, A.´ S´anchez-Monge2, L. Spinoglio9, J. Tig´e17, S. P. Trevi~no-Morales18, and G. J. White19; 20 1 Cardiff School of Physics and Astronomy, Cardiff University, Queen's Buildings, The Parade, Cardiff, Wales, CF24 3AA, UK 2 I. Physik. Institut, University of Cologne, 50937 Cologne, Germany 3 Laboratoire d'Astrophysique de Bordeaux, Univ. Bordeaux, CNRS, B18N, all´eeG. Saint-Hilaire, 33615 Pessac, France 4 Universit´eGrenoble Alpes, CNRS, Institut de Planetologie et d'Astrophysique de Grenoble, 38000 Grenoble, France 5 Laboratoire AIM, CEA/IRFU { CNRS/INSU { Universit´eParis Diderot, CEA-Saclay, 91191 Gif-sur-Yvette Cedex, France 6 NRC, Herzberg Institute of Astrophysics, Victoria, Canada 7 Jeremiah Horrocks Institute, University of Central Lancashire, Preston PR1 2HE, UK 8 Department of Physics and Astronomy, West Virginia University, Morgantown, WV 26506, USA 9 INAF { Istituto di Astrofisica e Planetologia Spaziali, via Fosso del Cavaliere 100, I-00133 Roma, Italy 10 -
Data Delivery Document
The CygnusX Legacy Project Data Description: Delivery 1: July 2011 CygnusX team: Joseph L. Hora1 (PI), Sylvain Bontemps2, Tom Megeath3, Nicola Schneider4 , Frederique Motte4, Sean Carey5, Robert Simon6, Eric Keto1, Howard Smith1, Lori Allen7, Rob Gutermuth8, Giovanni Fazio1, Kathleen Kraemer9, Don Mizuno10, Stephan Price9, Joseph Adams11, Xavier Koenig12 1Harvard-Smithsonian Center for Astrophysics, 2Observatoire de Bordeaux, 3University of Toledo, 4CEA-Saclay, 5Spitzer Science Center, 6Universität zu Köln, 7NOAO, 8Smith College, 9Air Force Research Lab, 10Boston College, 11Cornell University, 12Goddard Space Flight Center Table of Contents 1. Project Summary ..................................................................................................................... 3 1.1 IRAC observations ........................................................................................................... 3 1.2 MIPS observations ........................................................................................................... 4 1.3 Data reduction .................................................................................................................. 5 1.3.1 IRAC reduction ......................................................................................................... 5 1.3.2 MIPS reduction ......................................................................................................... 7 2. Point Source Catalog.............................................................................................................. -
DSLR PHOTOMETRY: a Citizen Science Project Using a Consumer Camera to Contribute Scientific Data
DSLR PHOTOMETRY: A citizen science project using a consumer camera to contribute scientific data by Mike Durkin Photometry is one of many areas in astronomy where amateurs can make useful contributions. Other areas include astrometry, occultation timings, and recording high quality observations of solar system objects. There are also projects for “armchair astronomers”, such as Galaxy Zoo. What is Photometry? Photometry is the measurement and study of the brightness of objects In astronomy, photometry is used to measure the brightness of stars , supernovae, asteroids , etc. I will be talking mostly about measuring variable stars , which are stars that change brightness over time. By studying the how the brightness of objects change over time, it can help determine physical properties. LIGHT CURVE shows brightness changes over time Cepheids are a type of variable stars that fluctuate in brightness. There is a well defined relationship between brightness and the Period of the brightness variation. Cepheids were used to determine the distance to the Andromeda Galaxy and proved that the universe was much larger than just the Milky Way. Light Curve for an asteroid can be used to show rotational period. Light curve for eclipsing binary The light curve for an eclipsing binary can be used to determine properties such as the diameters, luminosities, and separation of the stars. Eclipsing binary star animation courtesy of Wikimedia Commons THIS SOUNDS LIKE STUFF FOR PROFESSIONAL ASTRONOMERS, WHAT GOOD CAN AMATEURS DO? There are a lot more amateurs than professionals Estimated total number of professional astronomers is 2,080 (U.S. Dept. of Labor, Bureau of Labor Statistics) Estimated total number of amateurs is at least 100,000 based on the circulation numbers of magazines. -
Discovery of a Wolf–Rayet Star Through Detection of Its Photometric Variability
The Astronomical Journal, 143:136 (6pp), 2012 June doi:10.1088/0004-6256/143/6/136 C 2012. The American Astronomical Society. All rights reserved. Printed in the U.S.A. DISCOVERY OF A WOLF–RAYET STAR THROUGH DETECTION OF ITS PHOTOMETRIC VARIABILITY Colin Littlefield1, Peter Garnavich2, G. H. “Howie” Marion3,Jozsef´ Vinko´ 4,5, Colin McClelland2, Terrence Rettig2, and J. Craig Wheeler5 1 Law School, University of Notre Dame, Notre Dame, IN 46556, USA 2 Physics Department, University of Notre Dame, Notre Dame, IN 46556, USA 3 Harvard-Smithsonian Center for Astrophysics, Cambridge, MA 02138, USA 4 Department of Optics, University of Szeged, Hungary 5 Astronomy Department, University of Texas, Austin, TX 78712, USA Received 2011 November 9; accepted 2012 April 4; published 2012 May 2 ABSTRACT We report the serendipitous discovery of a heavily reddened Wolf–Rayet star that we name WR 142b. While photometrically monitoring a cataclysmic variable, we detected weak variability in a nearby field star. Low- resolution spectroscopy revealed a strong emission line at 7100 Å, suggesting an unusual object and prompting further study. A spectrum taken with the Hobby–Eberly Telescope confirms strong He ii emission and an N iv 7112 Å line consistent with a nitrogen-rich Wolf–Rayet star of spectral class WN6. Analysis of the He ii line strengths reveals no detectable hydrogen in WR 142b. A blue-sensitive spectrum obtained with the Large Binocular Telescope shows no evidence for a hot companion star. The continuum shape and emission line ratios imply a reddening of E(B − V ) = 2.2–2.6 mag. -
Exoplanet Community Report
JPL Publication 09‐3 Exoplanet Community Report Edited by: P. R. Lawson, W. A. Traub and S. C. Unwin National Aeronautics and Space Administration Jet Propulsion Laboratory California Institute of Technology Pasadena, California March 2009 The work described in this publication was performed at a number of organizations, including the Jet Propulsion Laboratory, California Institute of Technology, under a contract with the National Aeronautics and Space Administration (NASA). Publication was provided by the Jet Propulsion Laboratory. Compiling and publication support was provided by the Jet Propulsion Laboratory, California Institute of Technology under a contract with NASA. Reference herein to any specific commercial product, process, or service by trade name, trademark, manufacturer, or otherwise, does not constitute or imply its endorsement by the United States Government, or the Jet Propulsion Laboratory, California Institute of Technology. © 2009. All rights reserved. The exoplanet community’s top priority is that a line of probeclass missions for exoplanets be established, leading to a flagship mission at the earliest opportunity. iii Contents 1 EXECUTIVE SUMMARY.................................................................................................................. 1 1.1 INTRODUCTION...............................................................................................................................................1 1.2 EXOPLANET FORUM 2008: THE PROCESS OF CONSENSUS BEGINS.....................................................2 -
Cygnus X-3 and the Case for Simultaneous Multifrequency
by France Anne-Dominic Cordova lthough the visible radiation of Cygnus A X-3 is absorbed in a dusty spiral arm of our gal- axy, its radiation in other spectral regions is observed to be extraordinary. In a recent effort to better understand the causes of that radiation, a group of astrophysicists, including the author, carried 39 Cygnus X-3 out an unprecedented experiment. For two days in October 1985 they directed toward the source a variety of instru- ments, located in the United States, Europe, and space, hoping to observe, for the first time simultaneously, its emissions 9 18 Gamma Rays at frequencies ranging from 10 to 10 Radiation hertz. The battery of detectors included a very-long-baseline interferometer consist- ing of six radio telescopes scattered across the United States and Europe; the Na- tional Radio Astronomy Observatory’s Very Large Array in New Mexico; Caltech’s millimeter-wavelength inter- ferometer at the Owens Valley Radio Ob- servatory in California; NASA’s 3-meter infrared telescope on Mauna Kea in Ha- waii; and the x-ray monitor aboard the European Space Agency’s EXOSAT, a sat- ellite in a highly elliptical, nearly polar orbit, whose apogee is halfway between the earth and the moon. In addition, gamma- Wavelength (m) ray detectors on Mount Hopkins in Ari- zona, on the rim of Haleakala Crater in Fig. 1. The energy flux at the earth due to electromagnetic radiation from Cygnus X-3 as a Hawaii, and near Leeds, England, covered function of the frequency and, equivalently, energy and wavelength of the radiation.