Affiliations
Total Page:16
File Type:pdf, Size:1020Kb
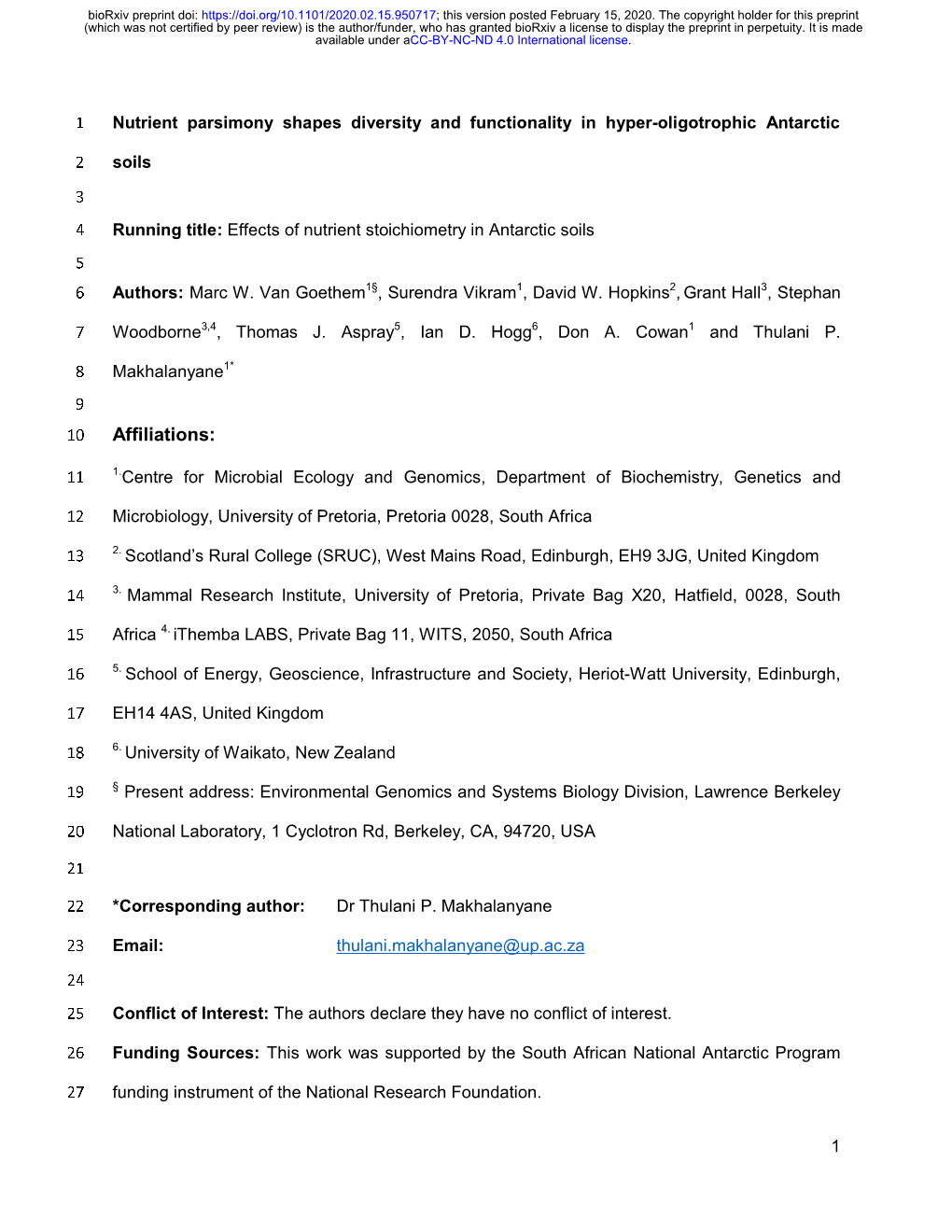
Load more
Recommended publications
-
A Primary Assessment of the Endophytic Bacterial Community in a Xerophilous Moss (Grimmia Montana) Using Molecular Method and Cultivated Isolates
Brazilian Journal of Microbiology 45, 1, 163-173 (2014) Copyright © 2014, Sociedade Brasileira de Microbiologia ISSN 1678-4405 www.sbmicrobiologia.org.br Research Paper A primary assessment of the endophytic bacterial community in a xerophilous moss (Grimmia montana) using molecular method and cultivated isolates Xiao Lei Liu, Su Lin Liu, Min Liu, Bi He Kong, Lei Liu, Yan Hong Li College of Life Science, Capital Normal University, Haidian District, Beijing, China. Submitted: December 27, 2012; Approved: April 1, 2013. Abstract Investigating the endophytic bacterial community in special moss species is fundamental to under- standing the microbial-plant interactions and discovering the bacteria with stresses tolerance. Thus, the community structure of endophytic bacteria in the xerophilous moss Grimmia montana were esti- mated using a 16S rDNA library and traditional cultivation methods. In total, 212 sequences derived from the 16S rDNA library were used to assess the bacterial diversity. Sequence alignment showed that the endophytes were assigned to 54 genera in 4 phyla (Proteobacteria, Firmicutes, Actinobacteria and Cytophaga/Flexibacter/Bacteroids). Of them, the dominant phyla were Proteobacteria (45.9%) and Firmicutes (27.6%), the most abundant genera included Acinetobacter, Aeromonas, Enterobacter, Leclercia, Microvirga, Pseudomonas, Rhizobium, Planococcus, Paenisporosarcina and Planomicrobium. In addition, a total of 14 species belonging to 8 genera in 3 phyla (Proteo- bacteria, Firmicutes, Actinobacteria) were isolated, Curtobacterium, Massilia, Pseudomonas and Sphingomonas were the dominant genera. Although some of the genera isolated were inconsistent with those detected by molecular method, both of two methods proved that many different endophytic bacteria coexist in G. montana. According to the potential functional analyses of these bacteria, some species are known to have possible beneficial effects on hosts, but whether this is the case in G. -
Taibaiella Smilacinae Gen. Nov., Sp. Nov., an Endophytic Member of The
International Journal of Systematic and Evolutionary Microbiology (2013), 63, 3769–3776 DOI 10.1099/ijs.0.051607-0 Taibaiella smilacinae gen. nov., sp. nov., an endophytic member of the family Chitinophagaceae isolated from the stem of Smilacina japonica, and emended description of Flavihumibacter petaseus Lei Zhang,1,2 Yang Wang,3 Linfang Wei,1 Yao Wang,1 Xihui Shen1 and Shiqing Li1,2 Correspondence 1State Key Laboratory of Crop Stress Biology for Arid Areas and College of Life Sciences, Xihui Shen Northwest A&F University, Yangling, Shaanxi 712100, PR China [email protected] 2State Key Laboratory of Soil Erosion and Dryland Farming on the Loess Plateau, Institute of Soil and Water Conservation, Chinese Academy of Sciences and Northwest A&F University, Yangling, Shaanxi 712100, PR China 3Hubei Institute for Food and Drug Control, Wuhan 430072, PR China A light-yellow-coloured bacterium, designated strain PTJT-5T, was isolated from the stem of Smilacina japonica A. Gray collected from Taibai Mountain in Shaanxi Province, north-west China, and was subjected to a taxonomic study by using a polyphasic approach. The novel isolate grew optimally at 25–28 6C and pH 6.0–7.0. Flexirubin-type pigments were produced. Cells were Gram-reaction-negative, strictly aerobic, rod-shaped and non-motile. Phylogenetic analysis based on 16S rRNA gene sequences showed that strain PTJT-5T was a member of the phylum Bacteroidetes, exhibiting the highest sequence similarity to Lacibacter cauensis NJ-8T (87.7 %). The major cellular fatty acids were iso-C15 : 0, iso-C15 : 1 G, iso-C17 : 0 and iso-C17 : 0 3-OH. -
Dinghuibacter Silviterrae Gen. Nov., Sp. Nov., Isolated from Forest Soil Ying-Ying Lv, Jia Wang, Mei-Hong Chen, Jia You and Li-Hong Qiu
International Journal of Systematic and Evolutionary Microbiology (2016), 66, 1785–1791 DOI 10.1099/ijsem.0.000940 Dinghuibacter silviterrae gen. nov., sp. nov., isolated from forest soil Ying-Ying Lv, Jia Wang, Mei-Hong Chen, Jia You and Li-Hong Qiu Correspondence State Key Laboratory of Biocontrol, School of Life Science, Sun Yat-sen University, Li-Hong Qiu Guangzhou, 510275, PR China [email protected] A novel Gram-stain negative, non-motile, rod-shaped, aerobic bacterial strain, designated DHOA34T, was isolated from forest soil of Dinghushan Biosphere Reserve, Guangdong Province, China. Comparative 16S rRNA gene sequence analysis showed that it exhibited highest similarity with Flavisolibacter ginsengiterrae Gsoil 492T and Flavitalea populi HY-50RT, at 90.89 and 90.83 %, respectively. In the neighbour-joining phylogenetic tree based on 16S rRNA gene sequences, DHOA34T formed an independent lineage within the family Chitinophagaceae but was distinct from all recognized species and genera of the family. T The major cellular fatty acids of DHOA34 included iso-C15 : 0, anteiso-C15 : 0, iso-C17 : 0 3-OH and summed feature 3 (C16 : 1v6c and/or C16 : 1v7c). The DNA G+C content was 51.6 mol% and the predominant quinone was menaquinone 7 (MK-7). Flexirubin pigments were produced. The phenotypic, chemotaxonomic and phylogenetic data demonstrate consistently that strain DHOA34T represents a novel species of a new genus in the family Chitinophagaceae, for which the name Dinghuibacter silviterrae gen. nov., sp. nov. is proposed. The type strain of Dinghuibacter silviterrae is DHOA34T (5CGMCC 1.15023T5KCTC 42632T). The family Chitinophagaceae, belonging to the class Sphingo- For isolation of DHOA34T, the soil sample was thoroughly bacteriia of the phylum Bacteroidetes, was proposed by suspended with 100 mM PBS (pH 7.0) and the suspension Ka¨mpfer et al. -
Affiliations
bioRxiv preprint doi: https://doi.org/10.1101/2020.02.15.950717; this version posted February 15, 2020. The copyright holder for this preprint (which was not certified by peer review) is the author/funder, who has granted bioRxiv a license to display the preprint in perpetuity. It is made available under aCC-BY-NC-ND 4.0 International license. 1 Nutrient parsimony shapes diversity and functionality in hyper-oligotrophic Antarctic 2 soils 3 4 Running title: Effects of nutrient stoichiometry in Antarctic soils 5 6 Authors: Marc W. Van Goethem1§, Surendra Vikram1, David W. Hopkins2, Grant Hall3, Stephan 7 Woodborne3,4, Thomas J. Aspray5, Ian D. Hogg6, Don A. Cowan1 and Thulani P. 8 Makhalanyane1* 9 10 Affiliations: 11 1.Centre for Microbial Ecology and Genomics, Department of Biochemistry, Genetics and 12 Microbiology, University of Pretoria, Pretoria 0028, South Africa 13 2. Scotland’s Rural College (SRUC), West Mains Road, Edinburgh, EH9 3JG, United Kingdom 14 3. Mammal Research Institute, University of Pretoria, Private Bag X20, Hatfield, 0028, South 15 Africa 4. iThemba LABS, Private Bag 11, WITS, 2050, South Africa 16 5. School of Energy, Geoscience, Infrastructure and Society, Heriot-Watt University, Edinburgh, 17 EH14 4AS, United Kingdom 18 6. University of Waikato, New Zealand 19 § Present address: Environmental Genomics and Systems Biology Division, Lawrence Berkeley 20 National Laboratory, 1 Cyclotron Rd, Berkeley, CA, 94720, USA 21 22 *Corresponding author: Dr Thulani P. Makhalanyane 23 Email: [email protected] 24 25 Conflict of Interest: The authors declare they have no conflict of interest. 26 Funding Sources: This work was supported by the South African National Antarctic Program 27 funding instrument of the National Research Foundation. -
Abstract Tracing Hydrocarbon
ABSTRACT TRACING HYDROCARBON CONTAMINATION THROUGH HYPERALKALINE ENVIRONMENTS IN THE CALUMET REGION OF SOUTHEASTERN CHICAGO Kathryn Quesnell, MS Department of Geology and Environmental Geosciences Northern Illinois University, 2016 Melissa Lenczewski, Director The Calumet region of Southeastern Chicago was once known for industrialization, which left pollution as its legacy. Disposal of slag and other industrial wastes occurred in nearby wetlands in attempt to create areas suitable for future development. The waste creates an unpredictable, heterogeneous geology and a unique hyperalkaline environment. Upgradient to the field site is a former coking facility, where coke, creosote, and coal weather openly on the ground. Hydrocarbons weather into characteristic polycyclic aromatic hydrocarbons (PAHs), which can be used to create a fingerprint and correlate them to their original parent compound. This investigation identified PAHs present in the nearby surface and groundwaters through use of gas chromatography/mass spectrometry (GC/MS), as well as investigated the relationship between the alkaline environment and the organic contamination. PAH ratio analysis suggests that the organic contamination is not mobile in the groundwater, and instead originated from the air. 16S rDNA profiling suggests that some microbial communities are influenced more by pH, and some are influenced more by the hydrocarbon pollution. BIOLOG Ecoplates revealed that most communities have the ability to metabolize ring structures similar to the shape of PAHs. Analysis with bioinformatics using PICRUSt demonstrates that each community has microbes thought to be capable of hydrocarbon utilization. The field site, as well as nearby areas, are targets for habitat remediation and recreational development. In order for these remediation efforts to be successful, it is vital to understand the geochemistry, weathering, microbiology, and distribution of known contaminants. -
Draft Genome Sequence of the Cellulolytic Endophyte Chitinophaga Costaii A37T2T Diogo N
Proença et al. Standards in Genomic Sciences (2017) 12:53 DOI 10.1186/s40793-017-0262-2 SHORTGENOMEREPORT Open Access Draft genome sequence of the cellulolytic endophyte Chitinophaga costaii A37T2T Diogo N. Proença1, William B. Whitman2, Nicole Shapiro3, Tanja Woyke3, Nikos C. Kyrpides3 and Paula V. Morais1,4* Abstract Here we report the draft genome sequence of Chitinophaga costai A37T2T (=CIP 110584T, =LMG 27458T), which was isolated from the endophytic community of Pinus pinaster tree. The total genome size of C. costaii A37T2T is 5.07 Mbp, containing 4204 coding sequences. Strain A37T2T encoded multiple genes likely involved in cellulolytic, chitinolytic and lipolytic activities. This genome showed 1145 unique genes assigned into 109 Cluster of Orthologous Groups in comparison with the complete genome of C. pinensis DSM 2588T. The genomic information suggests the potential of the strain A37T2T to interact with the plant metabolism. As there are only a few bacterial genomes related to Pine Wilt Disease, this work provides a contribution to the field. Keywords: Chitinophaga costaii A37T2, Cellulase, Chitinase, Genome sequence Introduction Bursaphelenchus xylophilus colonization in pine trees The genus Chitinophaga belongs to the family Chtinipha- [4]. Here, we show the second genome of the genus gaceae (phylum Bacteroidetes) alongside with the genera Chitinophaga, a draft genome of Chitinophaga costaii Arachidicoccus, Asinibacterium, Balneola, Cnuella, Creno- A37T2T, previously isolated as endophyte of Pinus pin- talea, Ferruginibacter, Filimonas, Flaviaesturariibacter, aster affected by PWD [1]. Flavihumibacter, Flavisolibacter, Flavitalea, Gracilimonas, Heliimonas, Hydrotalea, Lacibacter, Niabella, Niastella, Organism information Parasediminibacterium, Parasegetibacter, Sediminibacter- Classification and features ium, Segetibacter, Taibaiella, Terrimonas, Thermoflavifi- The type strain A37T2T (=CIP 110584T =LMG lum and Vibriomonas. -
Genome-Based Taxonomic Classification Of
ORIGINAL RESEARCH published: 20 December 2016 doi: 10.3389/fmicb.2016.02003 Genome-Based Taxonomic Classification of Bacteroidetes Richard L. Hahnke 1 †, Jan P. Meier-Kolthoff 1 †, Marina García-López 1, Supratim Mukherjee 2, Marcel Huntemann 2, Natalia N. Ivanova 2, Tanja Woyke 2, Nikos C. Kyrpides 2, 3, Hans-Peter Klenk 4 and Markus Göker 1* 1 Department of Microorganisms, Leibniz Institute DSMZ–German Collection of Microorganisms and Cell Cultures, Braunschweig, Germany, 2 Department of Energy Joint Genome Institute (DOE JGI), Walnut Creek, CA, USA, 3 Department of Biological Sciences, Faculty of Science, King Abdulaziz University, Jeddah, Saudi Arabia, 4 School of Biology, Newcastle University, Newcastle upon Tyne, UK The bacterial phylum Bacteroidetes, characterized by a distinct gliding motility, occurs in a broad variety of ecosystems, habitats, life styles, and physiologies. Accordingly, taxonomic classification of the phylum, based on a limited number of features, proved difficult and controversial in the past, for example, when decisions were based on unresolved phylogenetic trees of the 16S rRNA gene sequence. Here we use a large collection of type-strain genomes from Bacteroidetes and closely related phyla for Edited by: assessing their taxonomy based on the principles of phylogenetic classification and Martin G. Klotz, Queens College, City University of trees inferred from genome-scale data. No significant conflict between 16S rRNA gene New York, USA and whole-genome phylogenetic analysis is found, whereas many but not all of the Reviewed by: involved taxa are supported as monophyletic groups, particularly in the genome-scale Eddie Cytryn, trees. Phenotypic and phylogenomic features support the separation of Balneolaceae Agricultural Research Organization, Israel as new phylum Balneolaeota from Rhodothermaeota and of Saprospiraceae as new John Phillip Bowman, class Saprospiria from Chitinophagia. -
Chitinophaga Dinghuensis Sp. Nov., Isolated from Soil Ying-Ying Lv,3 Jia Wang,3 Jia You and Li-Hong Qiu
International Journal of Systematic and Evolutionary Microbiology (2015), 65, 4816–4822 DOI 10.1099/ijsem.0.000653 Chitinophaga dinghuensis sp. nov., isolated from soil Ying-ying Lv,3 Jia Wang,3 Jia You and Li-hong Qiu Correspondence State Key Laboratory of Biocontrol, School of Life Sciences, Sun Yat-sen University, Guangzhou, Li-hong Qiu 510275, PR China [email protected] A Gram-reaction-negative, aerobic, non-motile bacterial strain, DHOC24T, was isolated from the forest soil of Dinghushan Biosphere Reserve, Guangdong Province, PR China. Strain DHOC24T underwent a shape change during the course of culture from long filamentous cells (10–3060.4–0.5 mm) at 2 days to coccobacilli (0.5–1.060.7–1.0 mm) at 15 days after inoculation. It grew optimally at 28–33 8C and pH 6.5–7.5.The major quinone of T strainDHOC24 was MK-7, the main fatty acids were iso-C15 : 0,C16 : 1v5c and iso-C17 : 0 3-OH and the DNA G+C content was 43.1 mol%. On the basis of 16S rRNA gene sequence analysis, the strain was found to be affiliated with members of the genus Chitinophaga, but was clearly separated from established species of the genus. Strain DHOC24T was most closely related to Chitinophaga jiangningensis JN53T (98.3 % 16S rRNA gene sequence similarity) and Chitinophaga terrae KP01T (97.9 %). DNA–DNA hybridization study showed relatively low relatedness values (32.1 %) of strain DHOC24T with C. jiangningensis JN53T. The phenotypic, chemotaxonomic and phylogenetic data showed that strain DHOC24T represents a novel species of the genus Chitinophaga, for which the name Chitinophaga dinghuensis sp. -
Mooreia Alkaloidigena Gen. Nov., Sp. Nov. and Catalinimonas Alkaloidigena Gen
International Journal of Systematic and Evolutionary Microbiology (2013), 63, 1219–1228 DOI 10.1099/ijs.0.043752-0 Mooreia alkaloidigena gen. nov., sp. nov. and Catalinimonas alkaloidigena gen. nov., sp. nov., alkaloid-producing marine bacteria in the proposed families Mooreiaceae fam. nov. and Catalimonadaceae fam. nov. in the phylum Bacteroidetes Eun Ju Choi, Deanna S. Beatty, Lauren A. Paul, William Fenical and Paul R. Jensen Correspondence Center for Marine Biotechnology and Biomedicine, Scripps Institution of Oceanography, University Paul R. Jensen of California San Diego, La Jolla, CA 92093-0204, USA [email protected] Bacterial strains CNX-216T and CNU-914T were isolated from marine sediment samples collected from Palmyra Atoll and off Catalina Island, respectively. Both strains were Gram- negative and aerobic and produce deep-orange to pink colonies and alkaloid secondary metabolites. Cells of strain CNX-216T were short, non-motile rods, whereas cells of strain CNU- 914T were short, curved rods with gliding motility. The DNA G+C contents of CNX-216T and CNU-914T were respectively 57.7 and 44.4 mol%. Strains CNX-216T and CNU-914T contained MK-7 as the predominant menaquinone and iso-C15 : 0 and C16 : 1v5c as the major fatty acids. Phylogenetic analyses revealed that both strains belong to the order Cytophagales in the phylum Bacteroidetes. Strain CNX-216T exhibited low 16S rRNA gene sequence identity (87.1 %) to the nearest type strain, Cesiribacter roseus 311T, and formed a well-supported lineage that is outside all currently described families in the order Cytophagales. Strain CNU-914T shared 97.6 % 16S rRNA gene sequence identity with ‘Porifericola rhodea’ N5EA6-3A2B and, together with ‘Tunicatimonas pelagia’ N5DB8-4 and four uncharacterized marine bacteria isolated as part of this study, formed a lineage that is clearly distinguished from other families in the order Cytophagales. -
Genome-Based Taxonomic Classification of Bacteroidetes
Lawrence Berkeley National Laboratory Recent Work Title Genome-Based Taxonomic Classification of Bacteroidetes. Permalink https://escholarship.org/uc/item/2fs841cf Journal Frontiers in microbiology, 7(DEC) ISSN 1664-302X Authors Hahnke, Richard L Meier-Kolthoff, Jan P García-López, Marina et al. Publication Date 2016 DOI 10.3389/fmicb.2016.02003 Peer reviewed eScholarship.org Powered by the California Digital Library University of California ORIGINAL RESEARCH published: 20 December 2016 doi: 10.3389/fmicb.2016.02003 Genome-Based Taxonomic Classification of Bacteroidetes Richard L. Hahnke 1 †, Jan P. Meier-Kolthoff 1 †, Marina García-López 1, Supratim Mukherjee 2, Marcel Huntemann 2, Natalia N. Ivanova 2, Tanja Woyke 2, Nikos C. Kyrpides 2, 3, Hans-Peter Klenk 4 and Markus Göker 1* 1 Department of Microorganisms, Leibniz Institute DSMZ–German Collection of Microorganisms and Cell Cultures, Braunschweig, Germany, 2 Department of Energy Joint Genome Institute (DOE JGI), Walnut Creek, CA, USA, 3 Department of Biological Sciences, Faculty of Science, King Abdulaziz University, Jeddah, Saudi Arabia, 4 School of Biology, Newcastle University, Newcastle upon Tyne, UK The bacterial phylum Bacteroidetes, characterized by a distinct gliding motility, occurs in a broad variety of ecosystems, habitats, life styles, and physiologies. Accordingly, taxonomic classification of the phylum, based on a limited number of features, proved difficult and controversial in the past, for example, when decisions were based on unresolved phylogenetic trees of the 16S rRNA gene sequence. Here we use a large collection of type-strain genomes from Bacteroidetes and closely related phyla for Edited by: assessing their taxonomy based on the principles of phylogenetic classification and Martin G. -
Streptomyces Strains Modulate Dynamics of Soil Bacterial
www.nature.com/scientificreports OPEN Streptomyces strains modulate dynamics of soil bacterial communities and their efcacy in disease suppression caused by Phytophthora capsici Sakineh Abbasi1, Ayme Spor2, Akram Sadeghi3* & Naser Safaie1* The responses of rhizosphere bacterial communities of Streptomyces (SS14 and IT20 stains) treated- pepper plants following inoculation by Phytophthora capsici (PC) was investigated using Illumina MiSeq sequencing. Distinct modulation of the bacteriome composition was found for PC samples with the highest relative abundance (RA) of Chitinophaga (22 ± 0.03%). The RA of several bacterial operational taxonomic units (OTUs) was afected and caused changes in alpha and beta-diversity measures. In IT20, the RA of Cyanobacteria was enriched compared to SS14 (72%) and control samples (47%). Phylotypes belonging to Devosia, Promicromonospora, Kribbella, Microbacterium, Amylocolatopsis, and Pseudomonas genera in the rhizosphere were positively responding against the pathogen. Our fndings show that the phosphate solubilizing strain IT20 has higher microbial community responders than the melanin-producing strain SS14. Also, positive interactions were identifed by comparing bacterial community profles between treatments that might allow designing synthetic bio-inoculants to solve agronomic problems in an eco-friendly way. Streptomyces species, Gram-positive flamentous bacteria, are the most abundant and possibly the most impor- tant Actinomycetes1. Plant growth-promoting (PGP) Streptomyces strains colonize the rhizosphere/plant root and they could have potential as a bio-inoculant against biotic and abiotic stress conditions through difer- ent mechanisms2,3. In a previous study, PGP and biocontrol activity of S. rochei strain Y28 against Fusarium oxysporum f. sp. lycopersici race 3 causal agent of tomato Fusarium wilt was reported2. -
Niche Partitioning of Bacterial Communities in Biological Crusts And
View metadata, citation and similar papers at core.ac.uk brought to you by CORE provided by E-space: Manchester Metropolitan University's Research Repository Niche partitioning of bacterial communities in biological crusts and soils under grasses, shrubs and trees in the Kalahari David R Elliott1*, Andrew D Thomas2, Stephen R Hoon1, Robin Sen1 1. School of Science & the Environment, Manchester Metropolitan University, Manchester, M1 5GD, U.K. 2. Department of Geography and Earth Science, Aberystwyth University, Llandinam Building, Penglais, Aberystwyth, SY23 3DB, U.K. * Corresponding author [email protected] Keywords: biological soil crust, 454 pyrosequencing, bacterial community, Kalahari Sand, carbon, vegetation Abstract The Kalahari of southern Africa is characterised by sparse vegetation interspersed with microbe- dominated biological soil crusts (BSC) which deliver a range of ecosystem services including soil stabilisation and carbon fixation. We characterised the bacterial communities of BSCs (0-1 cm depth) and the subsurface soil (1-2 cm depth) in an area typical of lightly grazed Kalahari rangelands, composed of grasses, shrubs, and trees. Our data add substantially to the limited amount of existing knowledge concerning BSC microbial community structure, by providing the first bacterial community analyses of both BSCs and subsurface soils of the Kalahari region based on a high throughput 16S ribosomal RNA gene sequencing approach. BSC bacterial communities were distinct with respect to vegetation type and soil depth, and varied in relation to soil carbon, nitrogen, and surface temperature. Cyanobacteria were predominant in the grass interspaces at the soil surface (0-1 cm) but rare in subsurface soils (1-2 cm depth) and under the shrubs and trees.