Luminescence and Scintillation Properties of Csi -- a Potential Cryogenic Scintillator
Total Page:16
File Type:pdf, Size:1020Kb
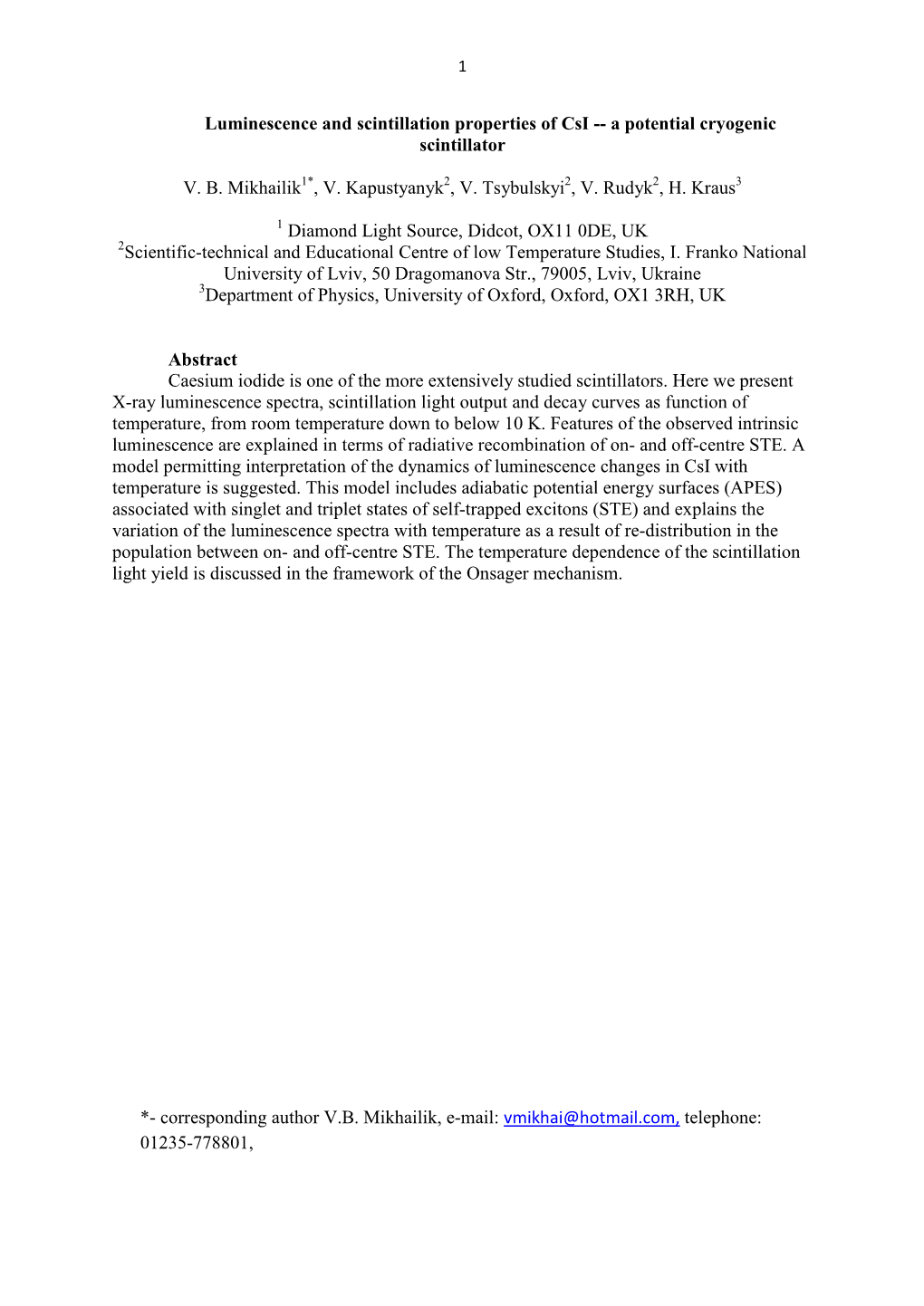
Load more
Recommended publications
-
ZEPLIN I—The UKDM Single Phase Xenon Experiment at Boulby Neil Spooner∗ Department of Physics and Astronomy University of Sheffield, Hounsfield Road, Sheffield, S3 7RH, UK
ZEPLIN I—The UKDM Single Phase Xenon Experiment at Boulby Neil Spooner∗ Department of Physics and Astronomy University of Sheffield, Hounsfield Road, Sheffield, S3 7RH, UK I briefly review the ZEPLIN I liquid xenon detector of the UKDM collaboration. 1. ZEPLIN I Detector The ZEPLIN I detector is a single phase, 3.6 kg fiducial mass, liquid xenon scintillation detector built by the UKDM Collaboration (RAL-Sheffield-ICSTM) and running at the Boulby underground site (see Figure 1). The target mass is housed in a Cu-101 copper vessel, shaped to maximise light collection, which provides a uniform temperature environment through the use of a cryo- liquid jacket. The fiducial volume of xenon is surrounded bya5mmPTFE reflector, giving diffuse scattering of the 175 nm scintillation photons to maximise light collection. This volume is viewed through 3 mm silica windows by three quartz windowed photomultipliers through optically isolated Œturrets¹ of liquid xenon. These act both as light guides and passive shielding for the X-ray emission from the photomultipliers. The novel use of the xenon turrets arose from experience with NaI detectors where solid high-grade silica is used for light guides and shields. However, the extensive use of such material for liquid xenon is precluded due to the absorption of the 175 nm photons. Scintillation light produced in the turret regions is seen predominantly by the nearest photomultiplier which allows the rejection of the photomultiplier X-ray events and the definition of a fiducial volume through a comparison of the light seen by each tube. Purification of the xenon is performed using Oxysorb ion exchange columns with additional purification by vacuum pumping on frozen xenon and subsequent fractionation of the xenon gas. -
Muon Decay 1
Muon Decay 1 LIFETIME OF THE MUON Introduction Muons are unstable particles; otherwise, they are rather like electrons but with much higher masses, approximately 105 MeV. Radioactive nuclear decays do not release enough energy to produce them; however, they are readily available in the laboratory as the dominant component of the cosmic ray flux at the earth’s surface. There are two types of muons, with opposite charge, and they decay into electrons or positrons and two neutrinos according to the rules + + µ → e νe ν¯µ − − µ → e ν¯e νµ . The muon decay is a radioactiveprocess which follows the usual exponential law for the probability of survival for a given time t. Be sure that you understand the basis for this law. The goal of the experiment is to measure the muon lifetime which is roughly 2 µs. With care you can make the measurement with an accuracy of a few percent or better. In order to achieve this goal in a conceptually simple way, we look only at those muons that happen to come to rest inside our detector. That is, we first capture a muon and then measure the elapsed time until it decays. Muons are rather penetrating particles, they can easily go through meters of concrete. Nevertheless, a small fraction of the muons will be slowed down and stopped in the detector. As shown in Figure 1, the apparatus consists of two types of detectors. There is a tank filled with liquid scintillator (a big metal box) viewed by two photomultiplier tubes (Left and Right) and two plastic scintillation counters (flat panels wrapped in black tape), each viewed by a photomul- tiplier tube (Top and Bottom). -
1. Gamma-Ray Detectors for Nondestructive Analysis P
1. GAMMA-RAY DETECTORS FOR NONDESTRUCTIVE ANALYSIS P. A. Russo and D. T. Vo I. INTRODUCTION AND OVERVIEW Gamma rays are used for nondestructive quantitative analysis of nuclear material. Knowledge of both the energy of the gamma ray and its rate of emission from the unknown mass of nuclear material is required to interpret most measurements of nuclear material quantities. Therefore, detection of gamma rays for nondestructive analysis of nuclear materials requires both spectroscopy capability and knowledge of absolute specific detector response. Some techniques nondestructively quantify attributes other than nuclear material mass, but all rely on the ability to distinguish elements or isotopes and measure the relative or absolute yields of their corresponding radiation signatures. All require spectroscopy and most require high resolution. Therefore, detection of gamma rays for quantitative nondestructive analysis (NDA) of the mass or of other attributes of nuclear materials requires spectroscopy. A previous book on gamma-ray detectors for NDA1 provided generic descriptions of three detector categories: inorganic scintillation detectors, semiconductor detectors, and gas-filled detectors. This report described relevant detector properties, corresponding spectral characteristics, and guidelines for choosing detectors for NDA. The current report focuses on significant new advances in detector technology in these categories. Emphasis here is given to those detectors that have been developed at least to the stage of commercial prototypes. The type of NDA application – fixed installation in a count room, portable measurements, or fixed installation in a processing line or other active facility (storage, shipping/receiving, etc.) – influences the choice of an appropriate detector. Some prototype gamma-ray detection techniques applied to new NDA approaches may revolutionize how nuclear materials are quantified in the future. -
Scintillation Detectors for X-Rays
INSTITUTE OF PHYSICS PUBLISHING MEASUREMENT SCIENCE AND TECHNOLOGY Meas. Sci. Technol. 17 (2006) R37–R54 doi:10.1088/0957-0233/17/4/R01 REVIEW ARTICLE Scintillation detectors for x-rays Martin Nikl Institute of Physics, Academy of Sciences of the Czech Republic, Cukrovarnicka 10, 162 53 Prague, Czech Republic Received 23 March 2005 Published 10 February 2006 Online at stacks.iop.org/MST/17/R37 Abstract Recent research in the field of phosphor and scintillator materials and related detectors is reviewed. After a historical introduction the fundamental issues are explained regarding the interaction of x-ray radiation with a solid state. Crucial parameters and characteristics important for the performance of these materials in applications, including the employed measurement methods, are described. Extended description of the materials currently in use or under intense study is given. Scintillation detector configurations are further briefly overviewed and selected applications are mentioned in more detail to provide an illustration. Keywords: luminescence intensity, luminescence kinetics, light detection, x-ray detection, scintillators, phosphors, traps and material imperfections (Some figures in this article are in colour only in the electronic version) 1. Introduction development of phosphor and scintillator materials to be used in their exploitation. It was 110 years ago in November 1895 that Wilhelm Conrad It is to be noticed that for registration of x-ray the so- Roentgen noticed the glow of a barium platino-cyanide screen, called direct registration principle is widely used, in which the placed next to his operating discharge tube, and discovered new incoming radiation is directly converted into electrical current invisible and penetrating radiation [1], which was named x-ray in a semiconducting material. -
2 & 4. %Z-E Cézé,26
Oct. 16, 1951 R. L. LONGIN 2,571,905 ZINC SULFIDE X-RAY PHOSFHORS Filed Aug. 28, 1947 777 (AZhosphor Afficiency) X (Kay Asorptiora Coefficieri.) Ahosphor Afficiency 2C-Alay Absorption Coefficier? . O ..f ..a 3 Azo de Araction of A Zux WITNESSES: INVENTOR 42% 42-az Aicha. d.?. longini. 2 & 4. %z-e RY Cézé,26. Patented Oct. 16, 1951 -3. 2,571,905 UNITED STATES PATENT office 2,571,905 ZINC SULFIDEX-RAY PHosPHORs Richard L. Longini, Pittsburgh, Pa., assignor to Westinghouse Electric Corporation, East Pitts burgh, Pa., a corporation of Pennsylvania, Application August 28, 1947, Serial No. 71,113 4 Claims. (C. 252-301.6) 1. 2 My invention relates to materials which be In making measurements of the luminous effi come fluorescent to produce visible light under ciency of Such materials, I have found that while the impact of X-rays and, in particular, relates Zinc sulphide produces a much larger yield of to a method of combining different substances visible radiation than does calcium tungstate for to produce a maximum yield of visible radia a given absorption of X-ray energy, the luminous tion for a given X-ray energization. intensity of the zinc sulphide screens is made In the medical and other X-ray arts, it is fre undesirably low because zinc sulphide has a very quently desirable to make visible the X-ray pat low absorption coefficient. I have, however, found terns produced by irradiating various objects that this difficulty can be corrected by admixing Which are opaque to visible radiation by streams 10 With the zinc sulphide an ancillary material or of X-rays, and screens covered by fluorescent ma “flux' in the form of an alkali halide. -
The Structure and Stability of Simple Tri-Iodides
THE STRUCTURE AND STABILITY OF SIMPLE TRI -IODIDES by ANTHONY JOHN THOMPSON FINNEY B.Sc.(Hons.) submitted in fulfilment of the requirements for the Degree of Doctor of Philosophy UNIVERSITY OF TASMANIA HOBART OCTOBER, 1973 . r " • f (i) Frontispiece (reproduced as Plate 6 - 1, Chapter 1) - two views of a large single crystal of KI 3 .H20. The dimensions of this specimen were approximately 3.0 cm x 1.5 cm x 0.5 cm. • - - . ;or • - This thesis contains no material which has been accepted for the award of any other degree or diploma in any University, and to the best of my knowledge and belief, this thesis contains no copy or paraphrase of material previously published or written by another person, except where reference is made in the text of this thesis. Anthony John Finney Contents page Abstract (iv) Acknowledgements (vii) Chapter 1 - The Structure and Stability of Simple 1 Tri-iodides Chapter 2 - The Theoretical Basis of X-Ray Structural 32 Analysis Chapter 3 - The Crystallographic Program Suite 50 Chapter 4 - The Refinement of the Structure of NH I 94 4 3 Chapter 5 - The Solution of the Structure of RbI 115 3 Chapter 6 - The Solution of the Structure of KI 3 .1120 135 Chapter 7 Discussion of the Inter-relation of 201 Structure and Stability Bibliography 255 Appendix A - Programming Details 267 Appendix B - Density Determinations 286 Appendix C - Derivation of the Unit Cell Constants of 292 KI .H 0 3 2 Appendix D - I -3 force constant Calculation 299 Appendix E - Publications 311 ( iv) THE STRUCTURE AND STABILITY OF SIMPLE TRI-IODIDES Abstract In this work the simple tri-iodides are regarded as being those in which the crystal lattice contains only cations, tri-iodide anions and possibly solvate molecules. -
Neutrino Detection with Liquid Scintillator Detectors
Neutrino Detection with Liquid Scintillator Detectors Minfang Yeh Neutrino and Nuclear Chemistry Chemistry/Instrumentation BNL VIRTUAL SYMPOSIUM FOR ETI/MTV CONSORTIA, 2021 Neutrino and Nuclear Chemistry at BNL since 1960+ 37 37 - HOMESTAKE 615t Cl + νe → Ar + e 71 71 - Gallex 30-t Ga + νe → Ge + e 780t D2O CC/NC SNO 200-kt H2O or 37-kt LAr LBNE (DUNE) 120-t 8% In-LS LENS 200-t 0.1% Gd-LS Daya Bay 780t 0.3% Nd/Te-LS SNO+ 6Li, 10B or Gd doped LS PROSPECT/LZ Metal-doped WbLS and 0νββ, dark-matter, AIT- plastic scintillator resins NEO, medical Water-based LS Scintillator Radiochemical Cerenkov 1960 1970 1980 1990 2000 2010 2020 M Yeh, BNL VIRTUAL SYMPOSIUM 2 LZ (Gd-doped) Dark Matter BNL-ν-Map SD, USA by liquid scintillator detectors AIT-NEO (WbLS) Boulby, UK JSNS2 (Gd-doped) SNO+ (Te-doped) Kamioka, Japan ANNIE Sudbury Canada (WbLS) BNL FNAL Daya Bay (Gd- doped), China PROSPECT (6Li-doped ), ORNL, TN, USA Cover a wide range of physics topics! neutrinos, dark matter, 0νββ, nonproliferation, medical physics,… M Yeh, BNL VIRTUAL SYMPOSIUM 3 Scintillation Mechanism S. Hans, J. Cumming, R. Rosero, S. Gokhale, R. Diaz, C. Camilo, M. Yeh, Light-yield quenching and remediation in liquid scintillator detectors, 2020 JINST 15 P12020 fast slow Stokes shift, photon-yield, timing structure, and C/H density determine the detector responses M Yeh, BNL VIRTUAL SYMPOSIUM 4 Scintillator Components C. Buck and M. Yeh, J. Phys. G: Nucl. Part. Phys. 43 093001 (2016) 200tons of Daya Bay Gd-LS produced in 2010; stable since production. -
Cosmic Ray Detector Hardware
Cosmic Ray Detector Hardware How it detects cosmic rays, what it measures and how to use it… Matthew Jones Purdue University 2012 QuarkNet Summer Workshop 1 What are Cosmic Rays? • Mostly muons down here… • Why are they called “rays”? – Purely historical • How can we detect them? – Muons are like heavy electrons – They have an electric charge – “Ionizing radiation”… – Some of their energy is transferred to the electrons in the material the move through – That’s what we detect… 2 Detecting Ionizing Radiation Cloud Chamber Solid State Geiger Counters Detectors Bubble Chamber Radiation creates Ion Chambers electron/hole pairs in Ionization initiates a silicon or germanium physical change in a that allow a current to gas or liquid. Wire Chambers flow. Photographic Film Crystal Scintillator GEM Detectors Photographic Organic An electric field does Emulsion Scintillator WORK on ionized gas Recombination of atoms to produce a Ionization initiates a electrons and ions voltage pulse. chemical reaction. produces light! 3 Plastic Scintillator 4 Plastic Scintillator • See, for example, Saint-Gobain, Inc. • Clear plastic traps light by total internal reflection. • Doped with a secret chemical that emits light when ionized, but does not re-absorb it. • Easy to cut, polish, bend, glue… • How much light is produced? – A muon travelling through 1 cm of plastic scintillator might produce about a thousand photons – Most of them would be blue – They bounce around inside the scintillator until they either escape or are absorbed • Usually wrapped in tin foil or white paper and then in black plastic or opaque paper to keep other light out. 5 The Cosmic Ray Detector Plastic scintillator wrapped in white paper and black plastic. -
Chemical Names and CAS Numbers Final
Chemical Abstract Chemical Formula Chemical Name Service (CAS) Number C3H8O 1‐propanol C4H7BrO2 2‐bromobutyric acid 80‐58‐0 GeH3COOH 2‐germaacetic acid C4H10 2‐methylpropane 75‐28‐5 C3H8O 2‐propanol 67‐63‐0 C6H10O3 4‐acetylbutyric acid 448671 C4H7BrO2 4‐bromobutyric acid 2623‐87‐2 CH3CHO acetaldehyde CH3CONH2 acetamide C8H9NO2 acetaminophen 103‐90‐2 − C2H3O2 acetate ion − CH3COO acetate ion C2H4O2 acetic acid 64‐19‐7 CH3COOH acetic acid (CH3)2CO acetone CH3COCl acetyl chloride C2H2 acetylene 74‐86‐2 HCCH acetylene C9H8O4 acetylsalicylic acid 50‐78‐2 H2C(CH)CN acrylonitrile C3H7NO2 Ala C3H7NO2 alanine 56‐41‐7 NaAlSi3O3 albite AlSb aluminium antimonide 25152‐52‐7 AlAs aluminium arsenide 22831‐42‐1 AlBO2 aluminium borate 61279‐70‐7 AlBO aluminium boron oxide 12041‐48‐4 AlBr3 aluminium bromide 7727‐15‐3 AlBr3•6H2O aluminium bromide hexahydrate 2149397 AlCl4Cs aluminium caesium tetrachloride 17992‐03‐9 AlCl3 aluminium chloride (anhydrous) 7446‐70‐0 AlCl3•6H2O aluminium chloride hexahydrate 7784‐13‐6 AlClO aluminium chloride oxide 13596‐11‐7 AlB2 aluminium diboride 12041‐50‐8 AlF2 aluminium difluoride 13569‐23‐8 AlF2O aluminium difluoride oxide 38344‐66‐0 AlB12 aluminium dodecaboride 12041‐54‐2 Al2F6 aluminium fluoride 17949‐86‐9 AlF3 aluminium fluoride 7784‐18‐1 Al(CHO2)3 aluminium formate 7360‐53‐4 1 of 75 Chemical Abstract Chemical Formula Chemical Name Service (CAS) Number Al(OH)3 aluminium hydroxide 21645‐51‐2 Al2I6 aluminium iodide 18898‐35‐6 AlI3 aluminium iodide 7784‐23‐8 AlBr aluminium monobromide 22359‐97‐3 AlCl aluminium monochloride -
Study of Electron Emissions of Some Mass Separated Fission Product Activities James Paul Adams Iowa State University
Iowa State University Capstones, Theses and Retrospective Theses and Dissertations Dissertations 1972 Study of electron emissions of some mass separated fission product activities James Paul Adams Iowa State University Follow this and additional works at: https://lib.dr.iastate.edu/rtd Part of the Nuclear Commons Recommended Citation Adams, James Paul, "Study of electron emissions of some mass separated fission product activities " (1972). Retrospective Theses and Dissertations. 5880. https://lib.dr.iastate.edu/rtd/5880 This Dissertation is brought to you for free and open access by the Iowa State University Capstones, Theses and Dissertations at Iowa State University Digital Repository. It has been accepted for inclusion in Retrospective Theses and Dissertations by an authorized administrator of Iowa State University Digital Repository. For more information, please contact [email protected]. INFORMATION TO USERS This dissertation was produced from a microfilm copy of the original document. While the most advanced technological means to photograph and reproduce this document have been used, the quality is heavily dependent upon the quality of the original submitted. The following explanation of techniques is provided to help you understand markings or patterns which may appear on this reproduction. 1. The sign or "target" for pages apparently lacking from the document photographed is "Missing Page(s)". If it was possible to obtain the missing page(s) or section, they are spliced into the film along with adjacent pages. This may have necessitated cutting thru an image and duplicating adjacent pages to insure you complete continuity. 2. When an image on the film is obliterated with a large round black mark, it is an indication that the photographer suspected that the copy may have moved during exposure and thus cause a blurred image. -
Durham E-Theses
Durham E-Theses Halogen and interhalogen adducts of substituted amido-ions Britton, G.C. How to cite: Britton, G.C. (1979) Halogen and interhalogen adducts of substituted amido-ions, Durham theses, Durham University. Available at Durham E-Theses Online: http://etheses.dur.ac.uk/8946/ Use policy The full-text may be used and/or reproduced, and given to third parties in any format or medium, without prior permission or charge, for personal research or study, educational, or not-for-prot purposes provided that: • a full bibliographic reference is made to the original source • a link is made to the metadata record in Durham E-Theses • the full-text is not changed in any way The full-text must not be sold in any format or medium without the formal permission of the copyright holders. Please consult the full Durham E-Theses policy for further details. Academic Support Oce, Durham University, University Oce, Old Elvet, Durham DH1 3HP e-mail: [email protected] Tel: +44 0191 334 6107 http://etheses.dur.ac.uk HALOGEN AND INTERHALOGEN ABDUCTS OF SUBSTITUTED AMI DO-IONS A THESIS PRESENTED BY G. C. BRITTON FOR THE DEGREE OF MASTER OF SCIENCE" OF THE UNIVERSITY OF DURHAM DEPARTMENT OF CHEMISTRY UNIVERSITY OF DURHAM The copyright of this thesis rests with the author. JUNE 1979 No quotation from it should be published without Unive. his prior written consent and information derived !*• SCIENCE "> 2 9 NOV V) from it should be acknowledged. SECTION LibraH ABSTRACT The reaction between N-dimethylchloramine and iodomethane - + which yields the species (CHj)4N (CH^)2N(lCl)2~ - has been investigated with a view to elucidating possible mechanisms, and a number of analagous and related compounds have been prepared. -
Download Article (PDF)
An X-Ray Diffraction Study on the Structure of Concentrated Aqueous Caesium Iodide and Lithium Iodide Solutions Yusuke Tamura, Toshio Yamaguchi *, Isao Okada. and Hitoshi Ohtaki Department of Electronic Chemistry, Tokyo Institute of Technology, Nagatsuta, Midori-ku, Yokohama 227, Japan Z. Naturforsch. 42 a, 367-376 (1987); received December 8, 1986 X-Ray scattering measurements of 2.78 and 5.56 molal aqueous solutions of caesium iodide and 2.78 and 6.05 molal lithium iodide were carried out at 293 and 343 K Differences in the radial distribution functions (DRDFs) have been obtained between the caesium iodide and lithium iodide solutions of similar composition, the latter being taken as a reference for the data analysis of the former. The DRDFs show a peak arising from Cs-I contact-ion-pairs at 390 pm for all the caesium iodide solutions. The hydration structure of the caesium and iodide ions has been revealed. Effects of the concentration and temperature on the formation of ion-pairs and on the hydration structure of the ions are discussed. 1. Introduction cept Li+ increases. However, the above studies have given only indirect information with respect to The structure of electrolyte solutions, in partic- structural properties of hydrated ions. Recently, ular alkali halide solutions, has widely been in- Heinzinger et al. have obtained direct structural vestigated by means of X-ray and neutron diffrac- information on the effect of temperature and pres- tion [1] and Monte Carlo and molecular dynamics sure on the hydration shells of Li+ and I- and on the simulations [2], from which the structure and prop- bulk water by means of molecular dynamics (MD) erties of hydrated ions have been revealed.