Tel-00007675.Pdf
Total Page:16
File Type:pdf, Size:1020Kb
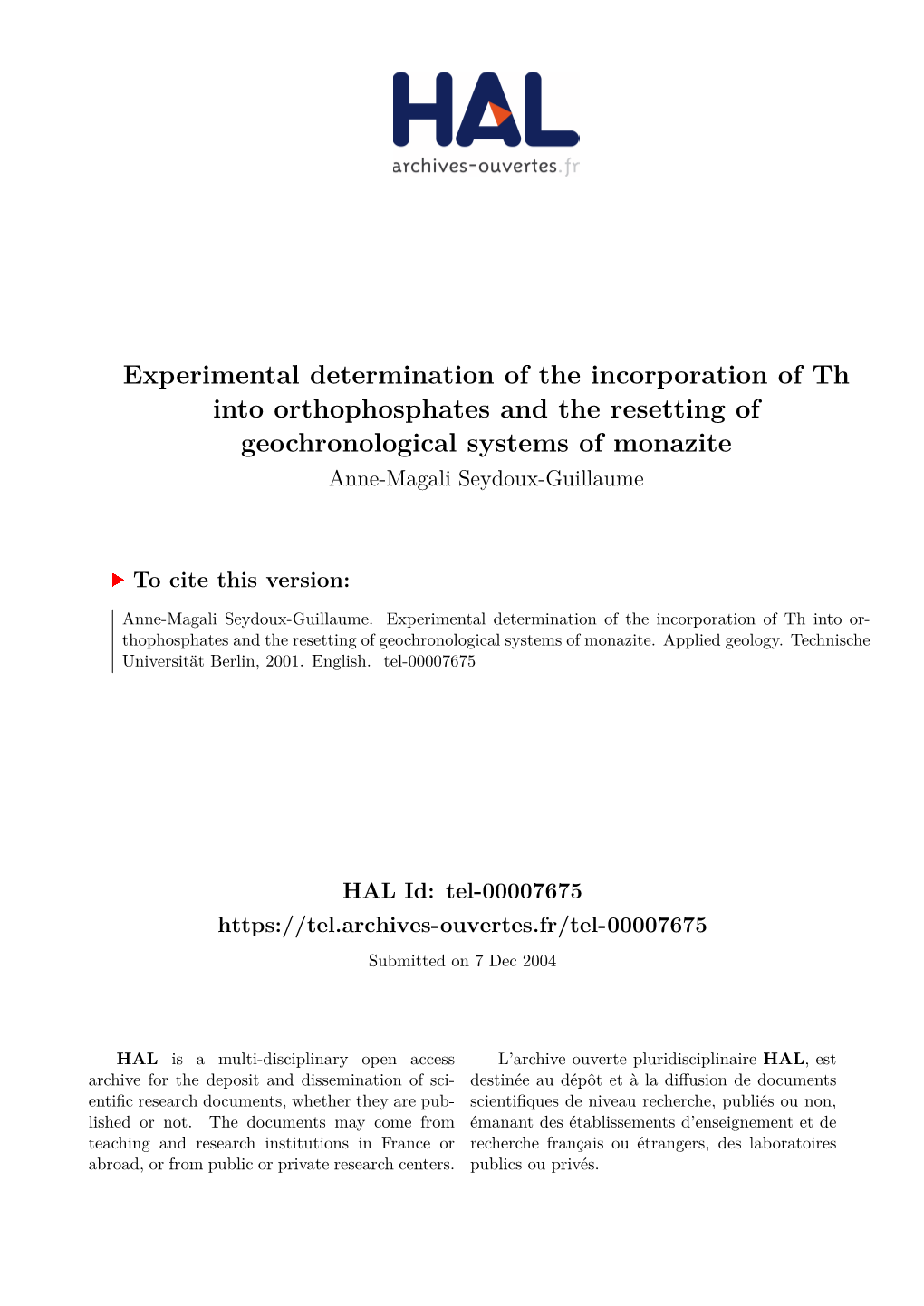
Load more
Recommended publications
-
Volcanic-Derived Placers As a Potential Resource of Rare Earth Elements: the Aksu Diamas Case Study, Turkey
minerals Article Volcanic-Derived Placers as a Potential Resource of Rare Earth Elements: The Aksu Diamas Case Study, Turkey Eimear Deady 1,*, Alicja Lacinska 2, Kathryn M. Goodenough 1, Richard A. Shaw 2 and Nick M. W. Roberts 3 1 The Lyell Centre, British Geological Survey, Research Avenue South, Edinburgh EH14 4AP, UK; [email protected] 2 Environmental Science Centre, British Geological Survey, Nicker Hill, Keyworth NG12 5GG, UK; [email protected] (A.L.); [email protected] (R.A.S.) 3 Environmental Science Centre, NERC Isotope Geosciences Laboratory, Nicker Hill, Keyworth NG12 5GG, UK; [email protected] * Correspondence: [email protected]; Tel.: +44-(0)131-6500217 Received: 15 February 2019; Accepted: 26 March 2019; Published: 30 March 2019 Abstract: Rare earth elements (REE) are essential raw materials used in modern technology. Current production of REE is dominated by hard-rock mining, particularly in China, which typically requires high energy input. In order to expand the resource base of the REE, it is important to determine what alternative sources exist. REE placers have been known for many years, and require less energy than mining of hard rock, but the REE ore minerals are typically derived from eroded granitic rocks and are commonly radioactive. Other types of REE placers, such as those derived from volcanic activity, are rare. The Aksu Diamas heavy mineral placer in Turkey has been assessed for potential REE extraction as a by-product of magnetite production, but its genesis was not previously well understood. REE at Aksu Diamas are hosted in an array of mineral phases, including apatite, chevkinite group minerals (CGM), monazite, allanite and britholite, which are concentrated in lenses and channels in unconsolidated Quaternary sands. -
Mineral Processing
Mineral Processing Foundations of theory and practice of minerallurgy 1st English edition JAN DRZYMALA, C. Eng., Ph.D., D.Sc. Member of the Polish Mineral Processing Society Wroclaw University of Technology 2007 Translation: J. Drzymala, A. Swatek Reviewer: A. Luszczkiewicz Published as supplied by the author ©Copyright by Jan Drzymala, Wroclaw 2007 Computer typesetting: Danuta Szyszka Cover design: Danuta Szyszka Cover photo: Sebastian Bożek Oficyna Wydawnicza Politechniki Wrocławskiej Wybrzeze Wyspianskiego 27 50-370 Wroclaw Any part of this publication can be used in any form by any means provided that the usage is acknowledged by the citation: Drzymala, J., Mineral Processing, Foundations of theory and practice of minerallurgy, Oficyna Wydawnicza PWr., 2007, www.ig.pwr.wroc.pl/minproc ISBN 978-83-7493-362-9 Contents Introduction ....................................................................................................................9 Part I Introduction to mineral processing .....................................................................13 1. From the Big Bang to mineral processing................................................................14 1.1. The formation of matter ...................................................................................14 1.2. Elementary particles.........................................................................................16 1.3. Molecules .........................................................................................................18 1.4. Solids................................................................................................................19 -
The Stability of Lead Isotopes from Thorium
MAY 24, I9I7] NATURE ---------------------------------------------------------245 but I Prof. Stefan lYle:yer may be I .have pointed out the unsuitability of makmg some exammatwn of the radiations of the thonum mmerals for age determination or correlation material, and the results he obtains will therefore be and this is particularly so i.n the case of minerals of very great value in deciding this point. the Palreozoic igneous rocks of Langesundfjord, Nor FREDERICK SoDDY. way. Mr. Lawson and myself based our former con Aberdeen, May 14. that lead could not be the end product of thonum largely on analyses of these minerals. How . PRO.F. SoDDY. having given me the privilege of read e:ver, I have recalculated the ratiQs on the assump mg h1s letter m advance, I should like to take the twn that thonum has one-seventh the lead-producing opportunity of directing attention to the geological age power of uranium, and it is satisfactory to find that :>f the thorium minerals of Ceylon, and to a few further when thorium is less than five times a·s abundant statistics bearing on the suggestion that only 35 per uranium, the ratios agree as closely on th'ts calculation cent. of thorium produces a stable isotope of lead. as do the simple lead-ratios. When thorium is more than five times as abundant as uranium neither set I a!ll to my friend, Mr. E. J. Wayland, late of ratios gives any approach to agreement although of Ceylon, for the follow mg prov1s1onal classdicat10n (in order of age) of the the minerals from anv one locality agree am'ong them older rocks of the island :- selves. -
Phase Decomposition Upon Alteration of Radiation-Damaged Monazite–(Ce) from Moss, Østfold, Norway
MINERALOGY CHIMIA 2010, 64, No. 10 705 doi:10.2533/chimia.2010.705 Chimia 64 (2010) 705–711 © Schweizerische Chemische Gesellschaft Phase Decomposition upon Alteration of Radiation-Damaged Monazite–(Ce) from Moss, Østfold, Norway Lutz Nasdala*a, Katja Ruschela, Dieter Rhedeb, Richard Wirthb, Ljuba Kerschhofer-Wallnerc, Allen K. Kennedyd, Peter D. Kinnye, Friedrich Fingerf, and Nora Groschopfg Abstract: The internal textures of crystals of moderately radiation-damaged monazite–(Ce) from Moss, Norway, indicate heavy, secondary chemical alteration. In fact, the cm-sized specimens are no longer mono-mineral monazite but rather a composite consisting of monazite–(Ce) and apatite pervaded by several generations of fractures filled with sulphides and a phase rich in Th, Y, and Si. This composite is virtually a ‘pseudomorph’ after primary euhedral monazite crystals whose faces are still well preserved. The chemical alteration has resulted in major reworking and decomposition of the primary crystals, with potentially uncontrolled elemental changes, including extensive release of Th from the primary monazite and local redeposition of radionuclides in fracture fillings. This seems to question the general alteration-resistance of orthophosphate phases in a low-temperature, ‘wet’ environment, and hence their suitability as potential host ceramics for the long-term immobilisation of ra- dioactive waste. Keywords: Chemical alteration · Monazite–(Ce) · Radiation damage · Thorium silicate 1. Introduction eventually to the formation of a non-crys- to undergo chemical alteration, and its in- talline form.[1,2] Such normally crystalline, crease with cumulative radiation damage, The accumulation of structural damage irradiation-amorphised minerals are com- ii) how exactly chemical alteration proc- generated by the corpuscular self-irra- monly described by the term ‘metamict’.[3] esses take place, and iii) as to which de- diation of minerals containing actinide The metamictisation process is controlled gree these materials (i.e. -
Raman Spectroscopic Study of Variably Recrystallized Metamict Zircon from Amphibolite-Facies Metagranites, Serbo-Macedonian Massif, Bulgaria
1357 The Canadian Mineralogist Vol. 44, pp. 1357-1366 (2006) RAMAN SPECTROSCOPIC STUDY OF VARIABLY RECRYSTALLIZED METAMICT ZIRCON FROM AMPHIBOLITE-FACIES METAGRANITES, SERBO-MACEDONIAN MASSIF, BULGARIA ROSITSA TITORENKOVA§ Central Laboratory of Mineralogy and Crystallography, Bulgarian Academy of Sciences, Acad. G. Bonchev Street 107, 1113 Sofi a, Bulgaria BORIANA MIHAILOVA Mineralogisch-Petrographisches Institut, University of Hamburg, Grindelallee 48, D–20146 Hamburg. Germany LUDMIL KONSTANTINOV Central Laboratory of Mineralogy and Crystallography, Bulgarian Academy of Sciences, Acad. G. Bonchev Street 107, 1113 Sofi a, Bulgaria ABSTRACT We investigated zircon from high-grade metagranites of the Serbo-Macedonian Massif, in Bulgaria, by cathodoluminescence (CL), back-scattered-electron imaging, electron-microprobe analysis, and Raman microspectroscopy. The structural state in various zones was assessed using: (i) the position and width of the Raman peak near 1008 cm–1, (ii) the relative Raman intensity –1 of the symmetrical and anti-symmetrical SiO4 modes, (iii) the width of the peaks near 357 and 439 cm , and (iv) the occurrence of extra Raman scattering near 162, 509, 635 and 785 cm–1. The analyzed zones are divided into two main groups: (A) areas with a well-resolved Raman peak near 1008 cm–1, and (B) areas with a very weak Raman scattering near 1008 cm–1. Group B can be classifi ed into two subgroups: (B-i) dark zones in CL images, with a high concentration of uranium (up to 7000 ppm), and (B-ii) outermost bright zones in CL images with a concentration of U lower than that in the inner areas and commonly below the detection limit. -
Stillwellite-(Ce) (Ce, La, Ca)Bsio
Stillwellite-(Ce) (Ce; La; Ca)BSiO5 c 2001 Mineral Data Publishing, version 1.2 ° Crystal Data: Hexagonal. Point Group: 3: As °at rhombohedral crystals, to 4 cm, and massive. Twinning: Observed about [100]. Physical Properties: Cleavage: One imperfect. Fracture: Conchoidal. Hardness = 6.5 D(meas.) = 4.57{4.60 D(calc.) = 4.67 » Optical Properties: Transparent to translucent. Color: Red-brown to pale pink; colorless in thin section. Streak: White. Optical Class: Uniaxial (+) to biaxial (+). ! = 1.765{1.784 ² = 1.780{1.787 2V(meas.) = 0±{6± Cell Data: Space Group: P 31: a = 6.841{6.844 c = 6.700{6.702 Z = 3 X-ray Powder Pattern: Mary Kathleen mine, Australia. 3.43 (s), 2.96 (s), 2.13 (ms), 4.44 (m), 1.864 (m), 2.71 (mw), 2.24 (mw) Chemistry: (1) (2) (1) (2) (1) (2) SiO2 22.40 22.06 La2O3 27.95 19.12 MgO 0.06 UO2 0.22 Ce2O3 33.15 30.82 CaO 0.95 0.34 ThO2 5.41 Pr2O3 1.82 F 0.30 + B2O3 12.23 [13.46] Nd2O3 5.36 H2O 0.85 Al2O3 0.42 Sm2O3 0.34 H2O¡ 0.10 Y2O3 0.74 0.28 Fe2O3 0.18 P2O5 0.67 Total [100.00] [99.26] (1) Mary Kathleen mine, Australia; recalculated to 100.00% after removal of very small amounts of uraninite and apatite determined by separate analysis. (2) Vico volcano, near Vetralla, Italy; by electron microprobe, B2O3 calculated from stoichiometry, original total given as 99.23%; corresponds to (Ce0:50La0:31Nd0:08Th0:05Pr0:03Ca0:02Sm0:01)§=1:00B1:02Si0:97O5: Occurrence: Locally abundant as a metasomatic replacement of metamorphosed calcareous sediments (Mary Kathleen mine, Australia); in alkalic pegmatites in syenite in an alkalic massif (Dara-i-Pioz massif, Tajikistan). -
U-Th-Pb Zircon Geochronology by ID-TIMS, SIMS, and Laser Ablation ICP-MS: Recipes, Interpretations, and Opportunities
ÔØ ÅÒÙ×Ö ÔØ U-Th-Pb zircon geochronology by ID-TIMS, SIMS, and laser ablation ICP-MS: recipes, interpretations, and opportunities U. Schaltegger, A.K. Schmitt, M.S.A. Horstwood PII: S0009-2541(15)00076-5 DOI: doi: 10.1016/j.chemgeo.2015.02.028 Reference: CHEMGE 17506 To appear in: Chemical Geology Received date: 17 November 2014 Revised date: 15 February 2015 Accepted date: 20 February 2015 Please cite this article as: Schaltegger, U., Schmitt, A.K., Horstwood, M.S.A., U-Th-Pb zircon geochronology by ID-TIMS, SIMS, and laser ablation ICP-MS: recipes, interpreta- tions, and opportunities, Chemical Geology (2015), doi: 10.1016/j.chemgeo.2015.02.028 This is a PDF file of an unedited manuscript that has been accepted for publication. As a service to our customers we are providing this early version of the manuscript. The manuscript will undergo copyediting, typesetting, and review of the resulting proof before it is published in its final form. Please note that during the production process errors may be discovered which could affect the content, and all legal disclaimers that apply to the journal pertain. ACCEPTED MANUSCRIPT U-Th-Pb zircon geochronology by ID-TIMS, SIMS, and laser ablation ICP-MS: recipes, interpretations, and opportunities U. Schaltegger1, A. K. Schmitt2, M.S.A. Horstwood3 1Earth and Environmental Sciences, Department of Earth Sciences, University of Geneva, Geneva, Switzerland ([email protected]) 2Department of Earth, Planetary, and Space Sciences, University of California, Los Angeles, USA ([email protected]) -
Crystallographic Study of Uranium-Thorium Bearing Minerals in Tranomaro, South-East Madagascar
Journal of Minerals and Materials Characterization and Engineering, 2013, 1, 347-352 Published Online November 2013 (http://www.scirp.org/journal/jmmce) http://dx.doi.org/10.4236/jmmce.2013.16053 Crystallographic Study of Uranium-Thorium Bearing Minerals in Tranomaro, South-East Madagascar Frank Elliot Sahoa1, Naivo Rabesiranana1*, Raoelina Andriambololona1, Nicolas Finck2, Christian Marquardt2, Hörst Geckeis2 1Institut National des Sciences et Techniques Nucléaires (Madagascar-INSTN), Antananarivo, Madagascar 2Institute of Nuclear Waste and Disposal (INE), Karlsruhe Institute of Technology (KIT), Karlsruhe, Germany Email: *[email protected] Received September 20, 2013; revised October 21, 2013; accepted November 5, 2013 Copyright © 2013 Frank Elliot Sahoa et al. This is an open access article distributed under the Creative Commons Attribution Li- cense, which permits unrestricted use, distribution, and reproduction in any medium, provided the original work is properly cited. ABSTRACT Studies are undertaken to characterize the uranium and thorium minerals of south-east Madagascar. Seven selected uranothorianite bearing pyroxenites samples from old abandoned uranium quarries in Tranomaro, south Amboasary, Madagascar (46˚28'00"E, 24˚36'00"S) have been collected. To determine the mineral micro-structure, they were inves- tigated for qualitative identification of crystalline compounds by using X-ray powder diffraction analytical method (XRD). Results showed that the uranium and thorium compounds, as minor elements, were present in various crystal- line structures. Thorium, as thorianite, is present in a simple ThO2 cubic crystalline system, whereas the uranium com- ponent of the Tranomaro uranothorianite samples is oxide-based and is a mixture of complex oxidation states and crys- talline systems. Generally called uraninite, its oxide compounds are present in more than eight phases. -
A Study on Monazite from the Ebisu Mine, Gifu Prefecture
MINERALOGICAL JOURNAL VOL. No. 4, pp. 224-235 JUNE, 1958 A STUDY ON MONAZITE FROM THE EBISU MINE, GIFU PREFECTURE TOSHIO KATO MineralogicalInstitute, Universityof Tokyo ABSTRACT Monazite from the Ebisu mine, Gifu Prefecture has been studied. The monazite from Ebisu is of yellowish or dark brown colour, with sp. gr.: 5,20. The result of chemical analysis of the sample is as follows: P2O5 26.81, SiO2 2,24, Al,O3 0.36, ThO2 4.51, U3O8 0.21, Ce2O3 24.58, La2-O3 etc, 38.51, Fe2O3 0.48, CaO 0.86, PbO 0.026, ig. loss 0.91, total 99.49% Monazites from Ratnapura, Ceylon and Minas Geraias, Brazil are also analysed for com parison. The relationships between the cell dimensions, the relative abundances of rare earths and the ThO2 content are discussed. The chemi cal age of the Ebisu monazite is 108 m.y., while its thorium lead age on the basis of mass-spectrometric data is fifty million years, in accordance with the geological evidence. Introduction Neagi district, Gifu Prefecture, is one of the most famous localitiess of radioactive minerals in Japan. Recently the writer studied monazite from the Ebisu mine in the district, applying mass spectroscopic method and determined its absolute age. The ages of several radioactive minerals from Japan have been determined by the chemical method, which is simply based on the ratio, of total lead to uranium plus thorium in a mineral. This ratio will not give reliable age unless severe prerequisites are satisfied. Occurrence The Ebisu mine, Wada, Hirukawa village, Ena county, Gifu Pre fecture, is situated about 14 kilometers north of the Oi railway station T. -
Three Occurrences of High-Thorian Uraninite Near Easton, Pennsylvania*
THE AMERICAN MINERALOGIST, YOL. 42, NOVEMBER_DECEMBER 1957 THREE OCCURRENCES OF HIGH-THORIAN URANINITE NEAR EASTON, PENNSYLVANIA* Anruun M oNrcounnu, Laf ayette C oll,ege, Easton, P ennsylaanio. Assrnacr The geology, mineralogy and paragenesis of three occurrences of high-thorian uraninite in serpentine near Easton, Pa., are described. seven *-ray fluorescence analyses together with three corroborative chemical analyses show this mineral to be high-thorian uraninite rather than high-uranoan thorianite as supposed six samples contajn from about l5/o to 3570ThOr. At the Williams quarry hydrothermai alteration of much uraninite to a sequence of secondary uranium- and thorium-rich minerals occurred. Frondel,s Mineral C, thoro- gummite, then boltrvoodite and uranophane were formed during serpentinization of frac- tured dolomite-diopside-tremolite contact-metamorphosed rock. Supergene coatings, chiefly hydrous uranium silicates and including boltwoodite and uranophane, formed much later. At the reservoir site slight hldrothermal alteration oI uraninite to thorogummite occurred. At the Royal Green Marble co. quarry uraninite is found unaltered. At these localities fracturing or temperatures during later hydrothermal mineralization may have been of lower intensity. It is concluded that thorium-rich Easton uraninite does not alter readily to secondary minerals, except when subjected to severe deformation and concomitant attack by ser- pentinizing hydrothermal solutions. The Th, rJ and. zr of this contact-metasomatic uran- inite and the associated zircon originated in a granite-pegmatite magma rich in these elements, which intruded dolomitic rocks and through deformation while crystallizing yielded the hydrothermal solutions that deposited these minerals in those rocks. then serpentinized the rocks. INrnonucrroN High-thorian uraninite, regardeduntil now as high-uranoan thorian- ite,1occurs in serpentinerock at three localitiesjust north and northeast of Easton, Pennsylvania.The two earlier discoverieswere made around 1930by GeorgeW. -
Petrology and Mineralogy of the Mount Rosa Area, El Paso and Teller Counties, Colorado
THE AMERICAN MINERALOGIST, VOL. 5 1, MARCH-APRIL, 1966 PETROLOGY AND MINERALOGY OF THE MOUNT ROSA AREA, EL PASO AND TELLER COUNTIES, COLORADO. II. PEGMATITESI EuceNB B. Gnoss, CaliJornia Diris,ion of Mines and Geology San Fra nci sco, C alifor n i o AND E. Wu. IIerNnrcu, Department of Geologyand Mineralogy The Llniversity of Michigan, Ann Arbor, Michigan. Aesrnlcr Two contrasting swarms of pegmatite dikes occur intermingled in the Pikes Peak granite and in related alkalic granites in the Mount Rosa area, west of Colorado Springs. One is the older calc-alkalic Pikes Peak type; the younger is the alkalic Mount Rosa type. The accessory assemblage of the Mount Rosa quartz-microcline-riebeckite pegmatites consists chiefly of radioactive zircon, fluorite, astrophyllite, thorite, and lesser amounts of pyrochlore, rutile, niobian rutile, columbite, bertrandite and rare aluminofluorides. These dikes intrude both the Pikes Peak and its derivative fayaiite granites along north- east-trending fractures. These pegmatites contrast markedly with the coexisting Pikes Peak pegmatites, which are characterized by quartz and amazonite crystals, zircon, biotite, siderite, and minor topaz, allanite, cassiterite and genthelvite. The Pikes Peak type occurs as segregations and injected bodies only within Pikes Peak granite. Age determinations on zircons from pegmatites of the Mount Rosa type proved to be inconsistent, but ages determined by means of the Kao/Ara0 method on pegmatitic rie- beckite are consistent rvith geological evidence and age determinations on the granites, giving an age of 1040 my. INrnolucrroN Discoverl' of unusual minerals in pegmatitesof the St. Peters Dome district, Mount Rosa area,Colorado, attracted the attention of mineralo- gists as earlv as 1877 (Koenig, 1877). -
Thermodynamics of Formation of Coffinite, Usio4
Thermodynamics of formation of coffinite, USiO4 Xiaofeng Guoa,b, Stéphanie Szenknectc, Adel Mesbahc, Sabrina Labsd, Nicolas Clavierc, Christophe Poinssote, Sergey V. Ushakova, Hildegard Curtiusd, Dirk Bosbachd, Rodney C. Ewingf, Peter C. Burnsg, Nicolas Dacheuxc, and Alexandra Navrotskya,1 aPeter A. Rock Thermochemistry Laboratory and Nanomaterials in the Environment, Agriculture, and Technology Organized Research Unit, University of California, Davis, CA 95616; bEarth and Environmental Sciences Division, Los Alamos National Laboratory, Los Alamos, NM 87545; cInstitut de Chimie Séparative de Marcoule, UMR 5257, CNRS/CEA/Université Montpellier/Ecole Nationale Supérieure de Chimie de Montpellier, Site de Marcoule, 30207 Bagnols sur Cèze, France; dInstitute of Energy and Climate Research, Nuclear Waste Management, Forschungszentrum Jülich GmbH, 52425 Jülich, Germany; eCEA, Nuclear Energy Division, RadioChemistry & Processes Department, BP 17171, 30207 Bagnols sur Cèze, France; fDepartment of Geological and Environmental Sciences, School of Earth Sciences, Stanford University, Stanford, CA 94305; and gDepartment of Civil and Environmental Engineering and Earth Sciences, University of Notre Dame, Notre Dame, IN 46556 Contributed by Alexandra Navrotsky, April 20, 2015 (sent for review February 10, 2015) Coffinite, USiO4, is an important U(IV) mineral, but its thermody- The precipitation of USiO4 as a secondary phase should be namic properties are not well-constrained. In this work, two dif- favored in contact with silica-rich groundwater (21) [silica − ferent coffinite samples were synthesized under hydrothermal concentration >10 4 mol/L (22, 23)]. Natural coffinite samples conditions and purified from a mixture of products. The enthalpy are often fine-grained (4, 5, 8, 11, 13, 15, 24), due to the long of formation was obtained by high-temperature oxide melt solu- exposure to alpha-decay event irradiation (4, 6, 25, 26) and are tion calorimetry.