A Natural Resource Condition Assessment for Sequoia and Kings Canyon National Parks Appendix 12 – Intact Forests
Total Page:16
File Type:pdf, Size:1020Kb
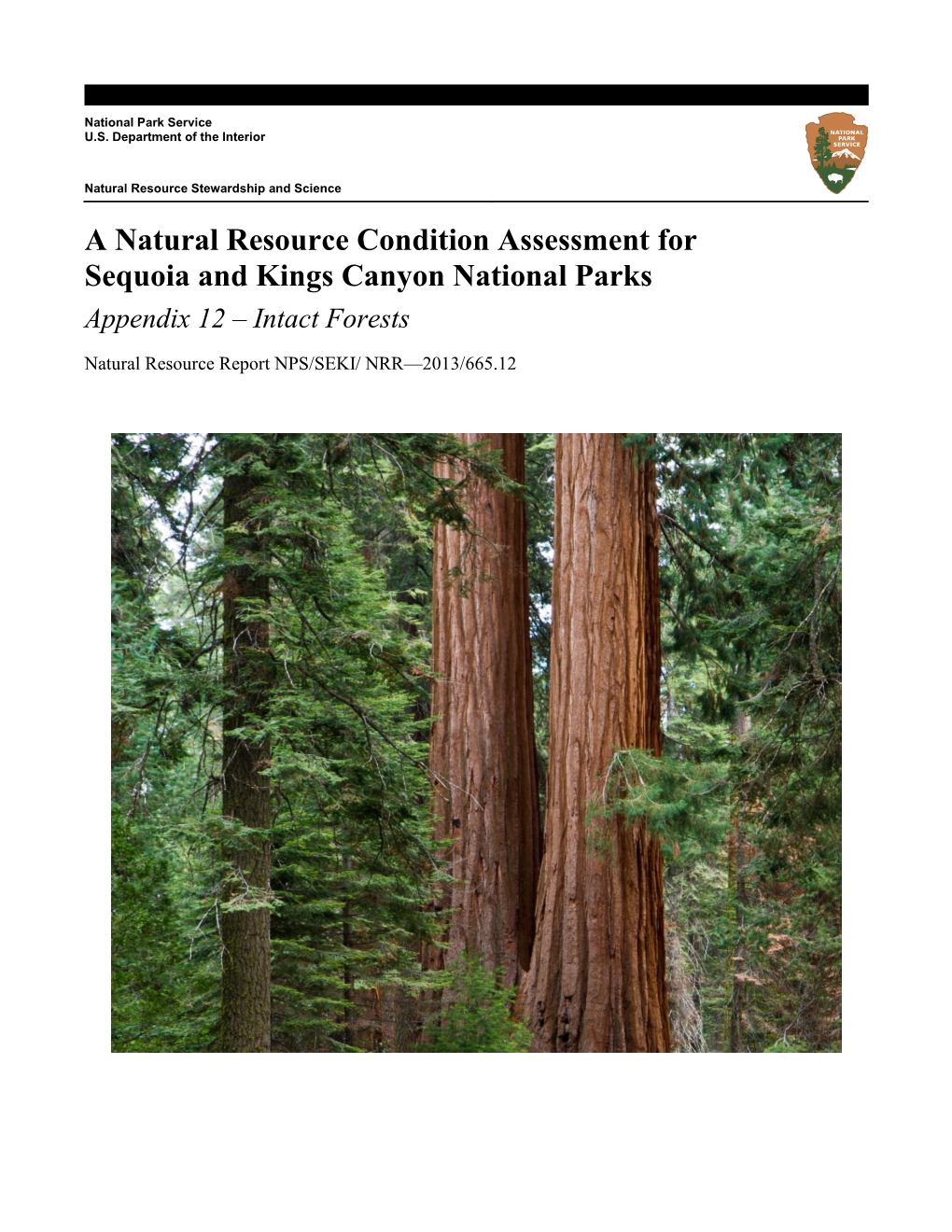
Load more
Recommended publications
-
Giant Sequoia National Monument, Draft Environmental Impact Statement Volume 1 1 Chapter 4 Environmental Consequences
United States Department of Giant Sequoia Agriculture Forest Service National Monument Giant Sequoia National Monument Draft Environmental Impact Statement August 2010 Volume 1 The U. S. Department of Agriculture (USDA) prohibits discrimination in all its programs and activities on the basis of race, color, national origin, gender, religion, age, disability, political beliefs, sexual orientation, or marital or family status. (Not all prohibited bases apply to all programs.) Persons with disabilities who require alternative means for communication of program information (Braille, large print, audiotape, etc.) should contact USDA’s TARGET Center at (202) 720-2600 (voice and TDD). To file a complaint of discrimination, write USDA, Director, Office of Civil Rights, Room 326-W, Whitten Building, 14th and Independence Avenue, SW, Washington, DC 20250-9410 or call (202) 720-5964 (voice and TDD). USDA is an equal opportunity provider and employer. Chapter 4 - Environmental Consequences Giant Sequoia National Monument, Draft Environmental Impact Statement Volume 1 1 Chapter 4 Environmental Consequences Volume 1 Giant Sequoia National Monument, Draft Environmental Impact Statement 2 Chapter 4 Environmental Consequences Chapter 4 Environmental Consequences Chapter 4 includes the environmental effects analysis. It is organized by resource area, in the same manner as Chapter 3. Effects are displayed for separate resource areas in terms of the direct, indirect, and cumulative effects associated with the six alternatives considered in detail. Effects can be neutral, beneficial, or adverse. This chapter also discusses the unavoidable adverse effects, the relationship between short-term uses and long-term productivity, and any irreversible and irretrievable commitments of resources. Environmental consequences form the scientific and analytical basis for comparison of the alternatives. -
Sequoia & Kings Canyon-Volume 1
Draft National Park Service U.S. Department of the Interior General Management Plan and Sequoia and Kings Canyon Comprehensive River Management Plan / National Parks Middle and South Forks of the Environmental Impact Statement Kings River and North Fork of the Kern River Tulare and Fresno Counties California Volume 1: Purpose of and Need for Action / The Alternatives / Index Page intentionally left blank SEQUOIA AND KINGS CANYON NATIONAL PARKS and MIDDLE AND SOUTH FORKS OF THE KINGS RIVER AND NORTH FORK OF THE KERN RIVER Tulare and Fresno Counties • California DRAFT GENERAL MANAGEMENT PLAN AND COMPREHENSIVE RIVER MANAGEMENT PLAN / ENVIRONMENTAL IMPACT STATEMENT Volume 1: Purpose of and Need for Action / The Alternatives / Index This document presents five alternatives that are being considered for the management and use of Sequoia and Kings Canyon National Parks over the next 15–20 years. The purpose of the Draft General Management Plan is to establish a vision for what Sequoia and Kings Canyon National Parks should be, including desired future conditions for natural and cultural resources, as well as for visitor experiences. The no-action alternative would continue current management direction, and it is the baseline for comparing the other alternatives (it was originally alternative B when the alternatives were first presented to the public in the winter of 2000). The preferred alternative is the National Park Service’s proposed action, and it would accommodate sustainable growth and visitor enjoyment, protect ecosystem diversity, and preserve basic character while adapting to changing user groups. Alternative A would emphasize natural ecosystems and biodiversity, with reduced use and development; alternative C would preserve the parks’ traditional character and retain the feel of yesteryear, with guided growth; and alternative D would preserve the basic character and adapt to changing user groups. -
Stock Users Guide to the Wilderness of Sequoia and Kings Canyon National Parks a Tool for Planning Stock-Supported Wilderness Trips
Sequoia & Kings Canyon National Park Service U.S. Department of the Interior National Parks Stock Users Guide to the Wilderness of Sequoia and Kings Canyon National Parks A tool for planning stock-supported wilderness trips SEQUOIA & KINGS CANYON NATIONAL PARKS Wilderness Office 47050 Generals Highway Three Rivers, California 93271 559-565-3766 [email protected] www.nps.gov/seki/planyourvisit/wilderness.htm Revised May 6th, 2021 EAST CREEK .............................................................................. 19 TABLE OF CONTENTS SPHINX CREEK .......................................................................... 19 INTRO TO GUIDE ........................................................................ 2 ROARING RIVER ....................................................................... 19 LAYOUT OF THE GUIDE............................................................. 3 CLOUD CANYON ....................................................................... 20 STOCK USE & GRAZING RESTRICTIONS: DEADMAN CANYON ................................................................ 20 KINGS CANYON NATIONAL PARK .................................... 4 SUGARLOAF AND FERGUSON CREEKS ................................. 21 SEQUOIA NATIONAL PARK ................................................ 6 CLOVER AND SILLIMAN CREEKS .......................................... 23 MINIMUM IMPACT STOCK USE ................................................ 8 LONE PINE CREEK .................................................................... 23 MINIMUM -
Gazetteer of Surface Waters of California
DEPARTMENT OF THE INTERIOR UNITED STATES GEOLOGICAL SURVEY GEORGE OTI8 SMITH, DIEECTOE WATER-SUPPLY PAPER 296 GAZETTEER OF SURFACE WATERS OF CALIFORNIA PART II. SAN JOAQUIN RIVER BASIN PREPARED UNDER THE DIRECTION OP JOHN C. HOYT BY B. D. WOOD In cooperation with the State Water Commission and the Conservation Commission of the State of California WASHINGTON GOVERNMENT PRINTING OFFICE 1912 NOTE. A complete list of the gaging stations maintained in the San Joaquin River basin from 1888 to July 1, 1912, is presented on pages 100-102. 2 GAZETTEER OF SURFACE WATERS IN SAN JOAQUIN RIYER BASIN, CALIFORNIA. By B. D. WOOD. INTRODUCTION. This gazetteer is the second of a series of reports on the* surf ace waters of California prepared by the United States Geological Survey under cooperative agreement with the State of California as repre sented by the State Conservation Commission, George C. Pardee, chairman; Francis Cuttle; and J. P. Baumgartner, and by the State Water Commission, Hiram W. Johnson, governor; Charles D. Marx, chairman; S. C. Graham; Harold T. Powers; and W. F. McClure. Louis R. Glavis is secretary of both commissions. The reports are to be published as Water-Supply Papers 295 to 300 and will bear the fol lowing titles: 295. Gazetteer of surface waters of California, Part I, Sacramento River basin. 296. Gazetteer of surface waters of California, Part II, San Joaquin River basin. 297. Gazetteer of surface waters of California, Part III, Great Basin and Pacific coast streams. 298. Water resources of California, Part I, Stream measurements in the Sacramento River basin. -
Late Summer Guide 2010
NATIONAL SEQUOIA & KINGS CANYON PARKS & SEQUOIA NATIONAL FORES T/ GIANT SEQUOIA NATIONAL MONUMENT LATE SUMMER GUIDE 2010 Crystal Cave / Free Activities • page 3 page 8 • Facilities & Ranger Programs in Sequoia Road Limits & Safety / Finding Gasoline • page 5 page 9 • Facilities & Programs in Kings Canyon & USFS Highlights & Shuttle in Sequoia Park • page 6 page 10 • Camping & Lodging / Bears & Your Food Highlights in Kings Canyon & USFS • page 7 page 12 • Expect Traffic Delays / Details & Park Map Discover • Protect • Connect Three verbs lie at the heart of a Classroom – helps to introduce great visit to your national parks: Sequoia and Kings Canyon to stu - discover the park for yourself, dents in some of the most under - connect to it on a personal level, served schools in the state. and choose to protect it! Rangers in the Classroom touches The number of people who can thousands of children each year. experience these parks this way is These parks lie just beyond their going up, thanks to a unique non- backyards, yet most of the kids have profit group. The Sequoia Parks never been here. Through curricu - Foundation raises funds for projects lum-based programs, they discover that make these parks easier to visit, a new world and start to see their via trails, exhibits, and classroom role in protecting it. They get excit - programs, to name just a few. ed about coming here with their A few examples of their projects: families and starting that personal • reworking trails to make them connection that can last a lifetime. more accessible for wheelchairs and Rangers travel as often as possi - anyone else who could use a ble to as many classrooms as they smoother walking surface; can – doing so in vans that were • support for trail-crew jobs that donated with help from the provide young people with experi - Foundation and other partners. -
Climbs and Expeditions, 1988
Climbs and Expeditions, 1988 The Editorial Board expresses its deep gratitude to the many people who have done so much to make this section possible. We cannot list them all here, but we should like to give particular thanks to the following: Kamal K. Guha, Harish Kapadia, Soli S. Mehta, H.C. Sarin, P.C. Katoch, Zafarullah Siddiqui, Josef Nyka, Tsunemichi Ikeda, Trevor Braham, Renato More, Mirella Tenderini. Cesar Morales Arnao, Vojslav Arko, Franci Savenc, Paul Nunn, Do@ Rotovnik, Jose Manuel Anglada, Jordi Pons, Josep Paytubi, Elmar Landes, Robert Renzler, Sadao Tambe, Annie Bertholet, Fridebert Widder, Silvia Metzeltin Buscaini. Luciano Ghigo, Zhou Zheng. Ying Dao Shui, Karchung Wangchuk, Lloyd Freese, Tom Elliot, Robert Seibert, and Colin Monteath. METERS TO FEET Unfortunately the American public seems still to be resisting the change from feet to meters. To assist readers from the more enlightened countries, where meters are universally used, we give the following conversion chart: meters feet meters feet meters feet meters feet 3300 10,827 4700 15,420 6100 20,013 7500 24,607 3400 11,155 4800 15,748 6200 20,342 7600 24,935 3500 11,483 4900 16,076 6300 20,670 7700 25,263 3600 11,811 5000 16,404 6400 20,998 7800 25,591 3700 12,139 5100 16,733 6500 21,326 7900 25,919 3800 12,467 5200 17.061 6600 21,654 8000 26,247 3900 12,795 5300 7,389 6700 21,982 8100 26,575 4000 13,124 5400 17,717 6800 22,3 10 8200 26,903 4100 13,452 5500 8,045 6900 22,638 8300 27,231 4200 13,780 5600 8,373 7000 22,966 8400 27,560 4300 14,108 5700 8,701 7100 23,294 8500 27,888 4400 14,436 5800 19,029 7200 23,622 8600 28,216 4500 14,764 5900 9,357 7300 23,951 8700 28,544 4600 15,092 6000 19,685 7400 24,279 8800 28,872 NOTE: All dates in this section refer to 1988 unless otherwise stated. -
Giant Sequoia National Monument, Draft Environmental Impact Statement Volume 1 1 Chapter 3 Affected Environment
United States Department of Giant Sequoia Agriculture Forest Service National Monument Giant Sequoia National Monument Draft Environmental Impact Statement August 2010 Volume 1 The U. S. Department of Agriculture (USDA) prohibits discrimination in all its programs and activities on the basis of race, color, national origin, gender, religion, age, disability, political beliefs, sexual orientation, or marital or family status. (Not all prohibited bases apply to all programs.) Persons with disabilities who require alternative means for communication of program information (Braille, large print, audiotape, etc.) should contact USDA’s TARGET Center at (202) 720-2600 (voice and TDD). To file a complaint of discrimination, write USDA, Director, Office of Civil Rights, Room 326-W, Whitten Building, 14th and Independence Avenue, SW, Washington, DC 20250-9410 or call (202) 720-5964 (voice and TDD). USDA is an equal opportunity provider and employer. Chapter 3 - Affected Environment Giant Sequoia National Monument, Draft Environmental Impact Statement Volume 1 1 Chapter 3 Affected Environment Volume 1 Giant Sequoia National Monument, Draft Environmental Impact Statement 2 Chapter 3 Affected Environment Chapter 3 Affected Environment Chapter 3 describes the affected environment or existing condition by resource area, as each is currently managed. This is the baseline condition against which environmental effects are evaluated and from which progress toward the desired condition can be measured. Vegetation, including Giant Sequoia Groves Vegetation within the Giant Sequoia National Monument can be grouped into ecological units with similar climatic, geology, soils, and vegetation communities. These units fall within three categories: oak woodlands/grasslands, shrublands/chaparral, and forestlands. The forested category between 5,000 and 7,000 feet in elevation, spanning the Monument from north to south, is dominated by mixed conifer and its variants. -
Sequoia En Kings Canyon National Park
Nationale Parken van Amerika @ www.ontdek-amerika.nl Last Update : Juli 6, 2015 Sequoia en Kings Canyon National Park KORTE OMSCHRIJVING Actuele Informatie Grootte : Sequoia NP = 1631 vierkante kilometer Kings Canyon NP = 1863 vierkante kilometer Hoogte : De hoogte van de wegen in het park varieert van 520 meter tot 2330 meter. Het hoogste punt is Mount Whitney. Deze 4418 meter hoge berg is de op één na hoogste berg van Amerika. Sequoia NP sinds : 25 september 1890 General Grant NP sinds : 1 oktober 1890 Kings Canyon NP sinds : 4 maart 1940 Sequoia en Kings Canyon zijn twee aan elkaar gelegen parken, die samen als één Nationaal Park worden beheerd. De meeste toeristen bezoeken het park vooral vanwege de aanwezigheid van de machtige Sequoiadendron; dit is qua volume de grootste boomsoort ter wereld. In de Sequoiabossen zijn veel eenvoudig begaanbare wandelpaden aangelegd. De bomen komen op diverse plaatsen in het park voor, maar de bossen beslaan samen maar een relatief klein deel ervan. In Kings Canyon National Park ligt een groot, onbedorven en werkelijk schitterend gedeelte van de Sierra Nevada Mountains. Er zijn diepe, door gletsjers uitgeslepen kloven, ontelbare meren, veel watervallen en bergweiden, en meer dan 20 rotspieken van rond de 4.000 meter hoogte. Het grootste deel van dit park is niet per auto toegankelijk, het zijn dan ook vooral doorgewinterde backpackers die dit gebied bezoeken. De drukst bezochte gebieden in Sequoia / Kings Canyon National Park zijn de sequoiabossen Giant Forest en Grant Grove; de kalksteengrot Crystal Cave en het ravijn Kings Canyon. In Giant Forest staat de General Sherman Tree, de grootste boom ter wereld. -
John Muir Papers
http://oac.cdlib.org/findaid/ark:/13030/kt709nf3b8 Online items available Register of the John Muir Papers Ronald H. Limbaugh, Kirsten E. Lewis, Don Walker, and Michael Wurtz Holt-Atherton Department of Special Collections University of the Pacific Library 3601 Pacific Ave. Stockton, CA 95211 Phone: (209) 946-2404 Fax: (209) 946-2942 URL: http://library.pacific.edu/ha © 2008 University of the Pacific. All rights reserved. Register of the John Muir Papers MSS 048 1 Register of the John Muir Papers Collection number: MSS 048 Holt-Atherton Department of Special Collections University of the Pacific Library Stockton, California Processed by: Ronald H. Limbaugh, Kirsten E. Lewis, Don Walker, and Michael Wurtz Date Completed: 2009 Encoded by: Michael Wurtz © 2008 University of the Pacific. All rights reserved. Descriptive Summary Title: John Muir papers Dates: 1849-1957 Collection number: MSS 048 Creator: Muir, John, 1838-1914 Collector: Muir-Hanna Trust Collection Size: 51 linear feet [6581 letters, 242 photographs, 384 drawings, and 78 journals are available online. ] Repository: University of the Pacific. Library. Holt-Atherton Dept. of Special Collections Stockton, California 95211 Abstract: The Muir Papers consists of John Muir's correspondence, journals, manuscripts, notebooks, drawings, and photographs. It also includes some Muir family papers, the William and Maymie Kimes collection of Muir's published writings, the Sierra Club Papers (1896-1913 ), materials collected and generated by his biographers William Badè and Linnie Marsh Wolf, and John Muir's clippings files and memorabilia. http://www.pacific.edu/Library/Find/Holt-Atherton-Special-Collections/Digital-Collections.html Shelf location: For current information on the location of these materials, please consult the library's online catalog. -
A Natural Resource Condition Assessment for Sequoia and Kings Canyon National Parks Appendix 15B - Animals of Conservation Concern, Supplemental Information
National Park Service U.S. Department of the Interior Natural Resource Stewardship and Science A Natural Resource Condition Assessment for Sequoia and Kings Canyon National Parks Appendix 15b - Animals of Conservation Concern, Supplemental Information Natural Resource Report NPS/SEKI/ NRR—2013/665.15b ON THE COVER Giant Forest, Sequoia National Park Photography by: Brent Paull A Natural Resource Condition Assessment for Sequoia and Kings Canyon National Parks Appendix 15b - Animals of Conservation Concern, Supplemental Information Natural Resource Report NPS/SEKI/ NRR—2013/665.15b The Ecology Graduate Student Project Collective (Jessica Blickley, Esther Cole, Stella Copeland, Kristy Deiner, Kristen Dybala, P. Bjorn Erickson, Rebecca Green, Katie Holzer, Chris Mosser, Erin Reddy, Meghan Skaer, Jamie Shields, Anna Steel, Zachary Steel, Evan Wolf) University of California, Davis Graduate Group in Ecology One Shields Avenue Davis, CA 95616 Mark W. Schwartz University of California, Davis John Muir Institute of the Environment One Shields Avenue Davis, CA 95616 June 2013 U.S. Department of the Interior National Park Service Natural Resource Stewardship and Science Fort Collins, Colorado The National Park Service, Natural Resource Stewardship and Science office in Fort Collins, Colorado, publishes a range of reports that address natural resource topics. These reports are of interest and applicability to a broad audience in the National Park Service and others in natural resource management, including scientists, conservation and environmental constituencies, and the public. The Natural Resource Report Series is used to disseminate high-priority, current natural resource management information with managerial application. The series targets a general, diverse audience, and may contain NPS policy considerations or address sensitive issues of management applicability. -
Onion Valley to Bishop Pass Below Are Very Tentative Day-By-Day Itineraries
Detailed Day by Day Description for Onion Valley to Bishop Pass Below are very tentative day-by-day itineraries. This can change for many reasons such as weather, minor injury, tired mules or unforeseen circumstances so please be flexible and go with any changes the backcountry dictates. Day 1 Onion Valley to Charlotte Lake 7.5 miles, 2870 ‘of gain, 1650 ‘of loss We enter the John Muir Wilderness at above Onion Valley and cross 11,850’ Kearsarge Pass before dropping to Char- lotte Lake, surely one of the most beautiful in the Sierra. Day 2 Charlotte Lake to Baxter Meadow 9.0 miles, 1825 ‘of gain, 2650 ‘of loss We will backtrack 1.3 miles to the John Muir Trail junction before turning north toward the narrow ridge of 11,978’ Glen Pass. The day will start in the trees, but soon climbs out of the shade to rocky slopes as it approaches the pass. You will pause at the top to celebrate the climb, but then linger to enjoy the spectacular views. Eventually we drop down from the pass for lunch at the stunning Rae Lakes and on past Arrowhead Lake to our camp by Baxter Meadow. Day 3 Layover at Baxter Meadow. Yes, it is early in the trip to be too tired and in need of a day of rest but this a beautiful place to stop and simply enjoy the Sierra Nevada or if the energy is high to walk to Baxter Pass or scramble one of the nearby peaks. Day 4 Baxter Meadow to Twin Lakes 9.1 miles, 2,100’ gain, 1,850’ loss We will spend the first half of the day walking 4.6 miles down a pleasant trail through forest and occasional meadows to Woods Creek. -
Sequoia National Forest Assessment Begins with This INTRODUCTION to Provide Background on the Process and to Describe the Assessment Area
CONTENTS INTRODUCTION ....................................................................................................................................................... 6 Purpose of the Assessment .................................................................................................................................. 6 Structure of the Assessment ................................................................................................................................ 6 Living Assessment ................................................................................................................................................... 7 Maps of the Assessment Area ............................................................................................................................. 8 Assessment Area, History and Distinctive Features ...................................................................................... 10 RESOURCES MANAGED AND EXISTING PLAN OBJECTIVES .................................................................... 12 BEST AVAILABLE SCIENTIFIC INFORMATION .............................................................................................. 16 FINDINGS ................................................................................................................................................................. 18 Chapter 1: Ecological Integrity .......................................................................................................................... 18 Important Information