Surface and Interfacial Design and Control of High Performing Thermoplastics: Polysulfones and Beyond
Total Page:16
File Type:pdf, Size:1020Kb
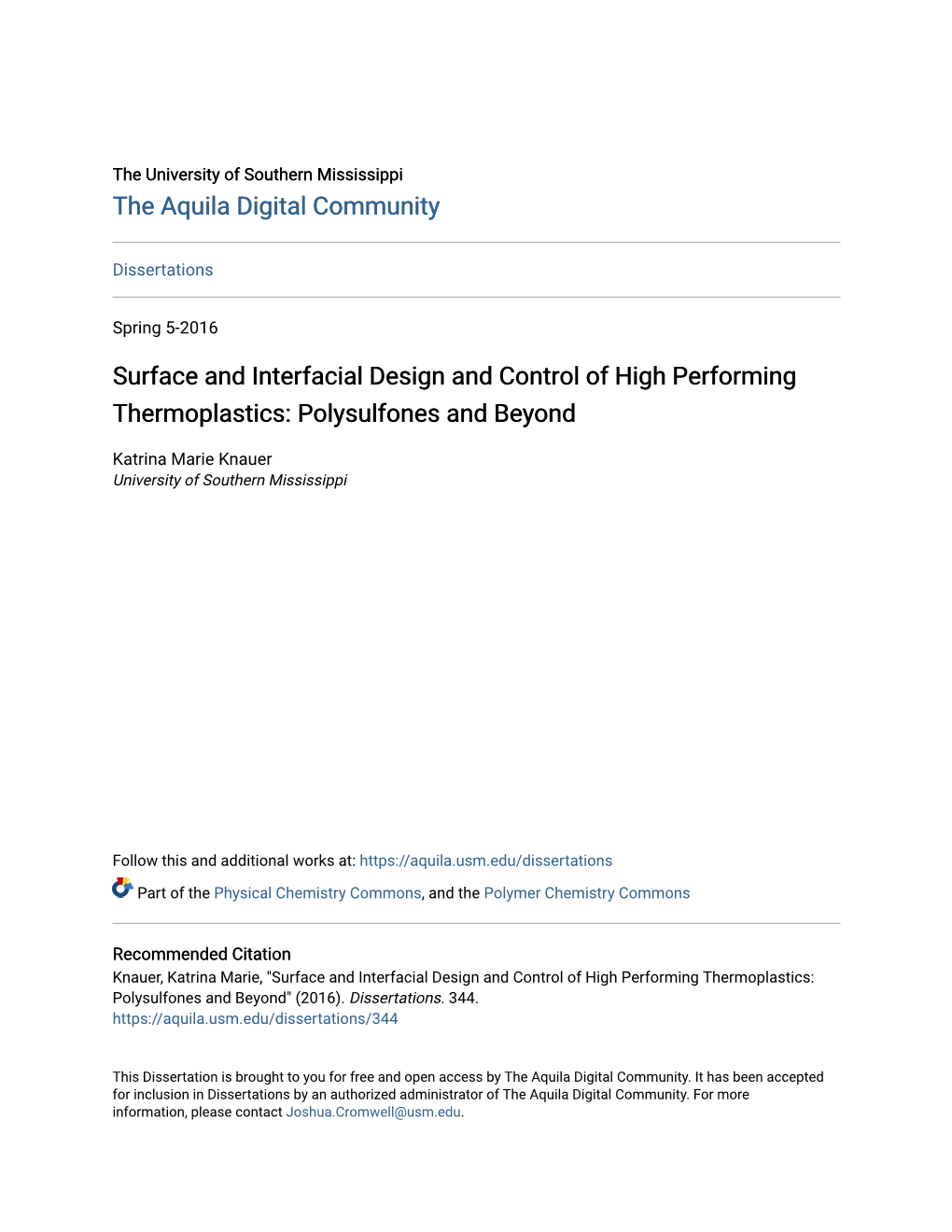
Load more
Recommended publications
-
Extrusion Foaming of Bioplastics for Lightweight Structure in Food Packaging
EXTRUSION FOAMING OF BIOPLASTICS FOR LIGHTWEIGHT STRUCTURE IN FOOD PACKAGING A thesis submitted for the degree of Doctor of Philosophy by Sitthi Duangphet School of Engineering and Design Brunel University December 2012 i Abstract This thesis reports the systematic approaches to overcome the key drawbacks of the pure PHBV, namely low crystallisation rate, tensile strength, ductility, melt viscosity, thermal stability and high materials cost. The physical, mechanical, thermal, and rheological properties of the pure PHBV were studied systematically first to lay a solid foundation for formulation development. The influence of blending with other biopolymers, inclusion of filler, and chain extender additives in terms of mechanical properties, rheology, thermal decomposition and crystallization kinetics were then followed. Creating lightweight structures by foaming is considered to be one of the effective ways to reduce material consumption, hence the reduction of density and morphology of PHBV-based foams using extrusion foaming technique were studied comprehensively in terms of extrusion conditions (temperature profiles, screw speed and material feeding rate) and the blowing agent content. The material cost reduction was achieved by adding low-cost filler (e.g. CaCO3) and reduction of density by foaming. The thermal instability was enhanced by incorporation of chain extender (e.g. Joncryl) and blending with a high thermal stability biopolymer (e.g. PBAT). The polymer blend also improved the ductility. Adding nucleation agent enhanced the crystallization rate to reduce stickiness of extruded sheet. The final formulation (PHBV/PBAT/CaCO3 composite) was successfully extruded into high quality sheet and thermoformed to produce prototype trays in an industrial scale trial. The effect of the extrusion conditions (temperature profiles, screw speed and material feeding rate) and the blowing agent content are correlated to the density reduction of the foams. -
Blown Films for Chilled and Frozen Food Packaging Applications
polymers Article Evaluation of the Suitability of Poly(Lactide)/Poly(Butylene-Adipate-co-Terephthalate) Blown Films for Chilled and Frozen Food Packaging Applications Arianna Pietrosanto, Paola Scarfato * , Luciano Di Maio , Maria Rossella Nobile and Loredana Incarnato Department of Industrial Engineering, University of Salerno, Via Giovanni Paolo II, 132, 84084 Fisciano (SA), Italy; [email protected] (A.P.); [email protected] (L.D.M.); [email protected] (M.R.N.); [email protected] (L.I.) * Correspondence: [email protected] Received: 20 December 2019; Accepted: 12 March 2020; Published: 3 April 2020 Abstract: The use of biopolymers can reduce the environmental impact generated by plastic materials. Among biopolymers, blends made of poly(lactide) (PLA) and poly(butylene-adipate-co-terephthalate) (PBAT) prove to have adequate performances for food packaging applications. Therefore, the present work deals with the production and the characterization of blown films based on PLA and PBAT blends in a wide range of compositions, in order to evaluate their suitability as chilled and frozen food packaging materials, thus extending their range of applications. The blends were fully characterized: they showed the typical two-phase structure, with a morphology varying from fibrillar to globular in accordance with their viscosity ratio. The increase of PBAT content in the blends led to a decrease of the barrier properties to oxygen and water vapor, and to an increase of the toughness of the films. The mechanical properties of the most ductile blends were also evaluated at 4 C and 25 C. The ◦ − ◦ decrease in temperature caused an increase of the stiffness and a decrease of the ductility of the films to a different extent, depending upon the blend composition. -
High Performance Ester Plasticizers
High Performance Ester Plasticizers Abstract Traditional elastomer polymers, such as nitrile, polychloroprene, chlorinated polyethylene and chlorosulfonated polyethylene, have for years used moderate- to low- performance ester plasticizers. However, longevity requirements for rubber articles made from these elastomers have created a need for higher-performance ester plasticizers. With the increasing high-temperature demands required by automotive, other elastomers such as acrylic, high-saturated nitrile, epichlorohydrin and ethylene propylene diene monomer EPDM are replacing the more traditional elastomers. Plasticizers commonly used for the traditional and the high-temperature polymers are extractable, incompatible or too volatile. This paper provides information on plasticizers that are designed for traditional elastomers and high-performance polymers. The test data will include heat aging, extraction by hydrocarbons and low-temperature as molded after aging. The information provided indicates that the permanence of the plasticizer after these various agings is the key to the retention of physical properties. Introduction End uses for elastomer compounds are quite diverse, but they can be loosely categorized as being either general performance or higher performance applications. Each of these performance categories requires a different set of considerations in terms of compounding with ester plasticizers. An ester plasticizer, in its simplest concept, is a high-boiling organic solvent that when added to an elastomeric polymer reduces stiffness and permits easier processing.1 For general performance applications, compounders require moderate performance in several areas without particular emphasis on any one. Some general performance ester plasticizers used in the marketplace today are DOA, DIDA, DIDP, DOP, DINP and other phthalates and adipates made from straight-chain alcohols of 7–11 carbons in length. -
A Glimpse of Biodegradable Polymers and Their Biomedical Applications
Spec. Matrices 2019; 7:1–19 Research Article Open Access Kazumasa Nomura* and Paul Terwilliger e-Polymers 2019; 19: 385–410 Self-dual Leonard pairs Review Article Open Access https://doi.org/10.1515/spma-2019-0001 Received May 8, 2018; accepted September 22, 2018 Tejas V. Shah and Dilip V. Vasava* Abstract: Let F denote a eld and let V denote a vector space over F with nite positive dimension. Consider A glimpse of biodegradablea pair A, A∗ of diagonalizable polymersF-linear maps and on V ,their each of which acts on an eigenbasis for the other one in an irreducible tridiagonal fashion. Such a pair is called a Leonard pair. We consider the self-dual case in which biomedical applicationsthere exists an automorphism of the endomorphism algebra of V that swaps A and A∗. Such an automorphism is unique, and called the duality A A∗. In the present paper we give a comprehensive description of this ↔ https://doi.org/10.1515/epoly-2019-0041 duality. In particular,NIPAM we - displayN-isopropylacrylamide an invertible F-linear map T on V such that the map X TXT− is the duality Received December 04, 2018; accepted March 29, 2019. → A A∗. We expressDEAMT -as N, a N-diethyl polynomial acrylamide in A and A∗. We describe how T acts on ags, decompositions, ↔ NVC - N-vinylcaprolactam Abstract: Over the past two decades, biodegradableand 24 bases for V. MVE - Methyl vinyl ether polymers (BPs) have been widely used in biomedical Keywords: LeonardPEO pair,- poly(ethylene tridiagonal matrix, oxide) self-dual applications such as drug carrier, gene delivery, tissue PPO - poly(propylene oxide) engineering, diagnosis, medical devices, and antibac- Classication: 17B37,15A21AAc - Acrylic acid terial/antifouling biomaterials. -
Bio-Based Food Packaging in Sustainable Development
Bio-based food packaging in Sustainable Development Challenges and opportunities to utilize biomass residues from agriculture and forestry as a feedstock for bio-based food packaging Author: Rubie van Crevel, intern Supervisor: Valeria Khristolyubova, Officer Forest Products Team Forestry Policy and Resources Division February 2016-June 2016 Table of contents Executive summary ......................................................................................................................... 4 1. Bio-based food packaging as a function of sustainable development ....................................... 7 2. Food packaging materials and environmental concerns .......................................................... 10 2.1 Methodology to assess environmental performances of a packaging material ................ 10 Life cycle thinking .................................................................................................................. 10 Life Cycle Assessment ........................................................................................................... 10 Limitations of Life Cycle Assessments .................................................................................. 12 2.2 Bio-based feedstock ............................................................................................................ 13 Defining bio-based products ................................................................................................. 13 Primary versus secondary biomass resources ..................................................................... -
Biodegradation and Hydrolysis Rate of Aliphatic Aromatic Polyester
Polymer Degradation and Stability 95 (2010) 2641e2647 Contents lists available at ScienceDirect Polymer Degradation and Stability journal homepage: www.elsevier.com/locate/polydegstab Biodegradation and hydrolysis rate of aliphatic aromatic polyester Thitisilp Kijchavengkul a, Rafael Auras a,*, Maria Rubino a, Susan Selke a, Mathieu Ngouajio b, R. Thomas Fernandez b a School of Packaging, Packaging Building, Michigan State University, East Lansing, MI 48824-1223, USA b Department of Horticulture, Plant & Soil Science Building, Michigan State University, East Lansing, MI 48824-1223, USA article info abstract Article history: The biodegradation and hydrolysis rates of an aliphatic aromatic copolyester were measured in manure, Received 20 April 2010 food, and yard compost environments and in phosphate buffer solution (pH ¼ 8.0) and vermiculite at Received in revised form 58 C. Mineralization, molecular weight reduction, and structural changes determined by DSC, FTIR, and 8 July 2010 1H NMR were used as indicators of the biodegradation and hydrolysis rates. Poly(butylene adipate- Accepted 26 July 2010 co-terephthalate), PBAT, film biodegraded at distinctive rates in manure, food, and yard compost envi- Available online 4 August 2010 ronments having different microbial activities. The highest biodegradation rate was found in manure compost, which had the highest CO emissions and lowest C/N ratio. The possible presence of extra- Keywords: 2 Mineralization cellular enzymes in manure and food composts may facilitate the hydrolytic reaction since greater 1 Poly(butylene adipate-co-terephthalate) molecular weight reduction rates were observed in these composts. H NMR and thermal analysis PBAT revealed that, while PBAT is a semi-crystalline copolyester with cocrystallization of BT and BA dimers, the Composting soft aliphatic domain (BA) and the amorphous region are more susceptible to hydrolysis and biodeg- radation than the rigid aromatic domain (BT) and the crystalline region. -
Environmental Impact of Food Packaging Materials: a Review of Contemporary Development from Conventional Plastics to Polylactic Acid Based Materials
materials Review Environmental Impact of Food Packaging Materials: A Review of Contemporary Development from Conventional Plastics to Polylactic Acid Based Materials Lindani Koketso Ncube 1, Albert Uchenna Ude 1,*, Enoch Nifise Ogunmuyiwa 2, Rozli Zulkifli 3 and Isaac Nongwe Beas 4 1 Department of Mechanical, Energy and Industrial Engineering, Faculty of Engineering and Technology (FET), Botswana International University of Science and Technology (BIUST), Private Mail Bag 16, Palapye, Botswana; [email protected] 2 Department of Chemical, Materials &Metallurgical Engineering Academic, FET, BIUST, Private Mail Bag 16, Palapye, Botswana; [email protected] 3 Department of Mechanical and Materials Engineering, Faculty of Engineering and Built Environment, The National University of Malaysia, UKM, Bangi 43600, Malaysia; rozlizulkifl[email protected] 4 Botswana Institute for Technology Research and Innovation (BITRI), Private Bag 0082, Gaborone, Botswana; [email protected] * Correspondence: [email protected] Received: 24 August 2020; Accepted: 28 September 2020; Published: 6 November 2020 Abstract: Plastics have remained the material of choice, and after serving their intended purpose, a large proportion ends up in the environment where they persist for centuries. The packaging industry is the largest and growing consumer of synthetic plastics derived from fossil fuels. Food packaging plastics account for the bulk of plastic waste that are polluting the environment. Additionally, given the fact that petroleum reserves are finite and facing depletion, there is a need for the development of alternative materials that can serve the same purpose as conventional plastics. This paper reviews the function of packaging materials and highlights the future potential of the adoption of green materials. -
And Poly(Butylene Adipate) - Quantitative Determination of Degradation Products and Application As PVC Plasticizers
Poly(butylene succinate) and Poly(butylene adipate) - Quantitative Determination of Degradation Products and Application as PVC Plasticizers Annika Lindström AKADEMISK AVHANDLING som med tillstånd av Kungliga Tekniska Högskolan framlägges till offentlig granskning för avläggande av teknisk licentiatexamen fredagen den 11 februari 2005, kl. 10.15 i Salongen, KTHB, Kungliga Tekniska Högskolan, Osquars backe 31, Stockholm. Avhandlingen försvaras på svenska. Abstract A solid phase extraction (SPE) method was developed for simultaneous extraction of dicarboxylic acids and diols formed during hydrolysis of poly(butylene succinate), PBS, and poly(butylene adipate), PBA. The developed SPE method and subsequent GC-MS analysis were used to extract, identify and quantify low molecular weight products migrating from linear and branched poly(butylene adipate) (PBA) and poly(butylene succinate) (PBS) during aging in aqueous media. The combination of SPE and GC-MS proved to be a sensitive tool, able to detect small differences in the degradation rate during early stages of hydrolysis before any significant differences were observed by weight loss and molecular weight measurements. The detected low molecular weight products included monomers i.e. adipic acid and 1,4-butanediol for the PBA polymers and succinic acid and 1,4-butanediol for PBS. Several dimers and trimers i.e. hydroxybutyl adipate, hydroxybutyl succinate, di(hydroxybutyl) adipate, di(hydroxybutyl) succinate and hydroxybutyl disuccinate were also detected. Best extraction efficiency for 1,4-butanediol and succinic acid was achieved with a hydroxylated polystyrene-divinylbenzene resin as solid phase. Linear range for the extracted analytes was 1-500 ng/µl for adipic acid and 2-500 ng/µl for 1,4-butanediol and succinic acid. -
(12) United States Patent (10) Patent No.: US 8,541,057 B2 Beatty (45) Date of Patent: Sep
US008541057B2 (12) United States Patent (10) Patent No.: US 8,541,057 B2 Beatty (45) Date of Patent: Sep. 24, 2013 (54) CYCLOHEXANEOXIDATION PROCESS (56) References Cited BYPRODUCT DERVATIVES AND METHODS FORUSING THE SAME U.S. PATENT DOCUMENTS 3,365.490 A 1/1968 Arthur et al. (75) Inventor: Richard P. Beatty, Newark, DE (US) 4,005,051 A * 1/1977 Brunner ........................ 524/310 4,273,179 A 6, 1981 Gardikes (73) Assignee: Invista North America S.A.R.L., 4,525,574 A 6, 1985 Altounian 5,981,769 A * 1 1/1999 Baur et al. .................... 549,266 Wilmington, DE (US) 6,008,418 A * 12/1999 Baur et al. ..... 568/853 6,288,286 B1* 9/2001 Stein et al. ............. ... 568,864 (*) Notice: Subject to any disclaimer, the term of this 6,313.358 B1 * 1 1/2001 Breitscheidel et al. ....... 568,864 patent is extended or adjusted under 35 6,407.294 B1* 6/2002 Breitscheidel et al. ....... 568,864 U.S.C. 154(b) by 124 days. 6,727,395 B1 * 4/2004 Stein et al. .................... 568,864 7,226,895 B2 6/2007 Xiang 2007/0112225 A1 5/2007 Sirch et al. .................... 568,864 (21) Appl. No.: 13/140,065 2008, 0207958 A1* 8, 2008 Haunert et al. 568,862 2009/0048471 A1* 2, 2009 Ii et al. .... 568,884 (22) PCT Filed: Dec. 11, 2009 2011/02O1848 A1* 8, 2011 Ii et al. ........... ... 568,833 2011/0263907 A1* 10, 2011 Abillard et al. 568/852 (86). PCT No.: PCT/US2O09/067634 2012/0101009 A1 * 4/2012 Beatty .......................... -
Review on the Impact of Polyols on the Properties of Bio-Based Polyesters
polymers Review Review on the Impact of Polyols on the Properties of Bio-Based Polyesters Kening Lang 1 , Regina J. Sánchez-Leija 1,2, Richard A. Gross 1,3,* and Robert J. Linhardt 1,3,4,* 1 Department of Chemistry and Chemical Biology and Center for Biotechnology and Interdisciplinary Studies, Rensselaer Polytechnic Institute, Troy, NY 12180, USA; [email protected] (K.L.); [email protected] (R.J.S.-L.) 2 Pritzker School of Molecular Engineering, The University of Chicago, 5640 S Ellis Ave, Chicago, IL 60637, USA 3 Department of Biomedical Engineering and Center for Biotechnology and Interdisciplinary Studies, Rensselaer Polytechnic Institute, Troy, NY 12180, USA 4 Department of Biology and Center for Biotechnology and Interdisciplinary Studies, Rensselaer Polytechnic Institute, Troy, NY 12180, USA * Correspondence: [email protected] (R.A.G.); [email protected] (R.J.L.); Tel.: +1-518-276-3734 (R.A.G.); +1-518-276-3404 (R.J.L.) Received: 25 November 2020; Accepted: 9 December 2020; Published: 12 December 2020 Abstract: Bio-based polyol polyesters are biodegradable elastomers having potential utility in soft tissue engineering. This class of polymers can serve a wide range of biomedical applications. Materials based on these polymers are inherently susceptible to degradation during the period of implantation. Factors that influence the physicochemical properties of polyol polyesters might be useful in achieving a balance between durability and biodegradability. The characterization of these polyol polyesters, together with recent comparative studies involving creative synthesis, mechanical testing, and degradation, have revealed many of their molecular-level differences. The impact of the polyol component on the properties of these bio-based polyesters and the optimal reaction conditions for their synthesis are only now beginning to be resolved. -
Use of Thermoplastic Starch in Poly(Lactic Acid)/Poly(Butylene Adipate-Co-Terephthalate) Based
Use of thermoplastic starch in poly(lactic acid)/poly(butylene adipate-co-terephthalate) based nanocomposites for bio-based food packaging by Pavan Harshit Manepalli B.Tech, Indian Institute of Technology, Kharagpur, 2012 M.S., Kansas State University, 2014 AN ABSTRACT OF A DISSERTATION submitted in partial fulfillment of the requirements for the degree DOCTOR OF PHILOSOPHY Department of Grain Science and Industry College of Agriculture KANSAS STATE UNIVERSITY Manhattan, Kansas 2019 Abstract Poly(lactic acid) (PLA) is the most common bio-based & compostable polymer available commercially that is cost competitive and combines a range of desirable properties like melt processability, high strength and modulus. The films made from this aliphatic polyester tend to be brittle which can be overcome by blending PLA with another bio-based polymer with high flexibility poly(butylene adipate-co-terephthalate) (PBAT), but the resultant blend is only biodegradable in composting conditions. The primary focus of this study was incorporation of thermoplastic starch (TPS) in PLA/PBAT blends to increase the rate of biodegradability and decrease the cost. In the first part of this study, as a preliminary step only PLA/PBAT blends were investigated along with nanofiller nanocrystalline cellulose (NCC) as a nanofiller for enhancing mechanical and barrier properties. Melt extrusion was used for preparation of nanocomposites and 200 microns thick films were formed by melt pressing. PBAT enhanced elongation but NCC did not have any positive impact on the mechanical and barrier properties of the nanocomposites as NCC was aggregated in the polymer matrix due to the difference in polarity based on the hydrophilic nature of the nanofiller and hydrophobic nature of the polymer matrix. -
Polymer-To-Solvent Reference Table for GPC/SEC
Polymer-to-Solvent Reference Table for GPC/SEC Technical Overview Author Abstract Adam Bivens, A comprehensive reference on solvents for gel permeation chromatography, with Agilent Technologies a full list of tested solvent, polymer, and column combinations, and notes on best practices. Introduction Experimental Each chemistry was formulated to be noninteractive to a wide variety of Gel permeation chromatography For GPC to yield valid data, the analytes polymers dissolved in their respective (GPC), which is also referred to as size must not stick to the surface of the solvents. Polymeric media allow for exclusion chromatography (SEC) or gel particle, as this would cause the retention both wide pores and a single, consistent fi ltration chromatography (GFC), provides time to no longer refl ect just size, but also surface chemistry free of interactive size-based separation by allowing surface interaction effects. silanols or metal centers found on other analytes with small size in solution materials. to diffuse into the pores of the media, To overcome this challenge, Agilent provides three phase chemistries that while analytes that are too large to fi t Over its 40-year history, Agilent has minimize surface interaction in their into these pores are swept through the assembled a vast library of applications respective solvents: column by the solvent. for GPC, which are summarized in Table 2. Pore size and distribution can • PLgel – A highly cross-linked be manipulated to change the polystyrene-divinylbenzene retention-to-size relationship and (PS-DVB) particle compatible with Table 1. Chemical acronyms. the range of separable sizes. This organic and many polar organic THF Tetrahydrofuran retention-to-size relationship is solvents.