Progress in Oceanography Progress in Oceanography 72 (2007) 39–62
Total Page:16
File Type:pdf, Size:1020Kb
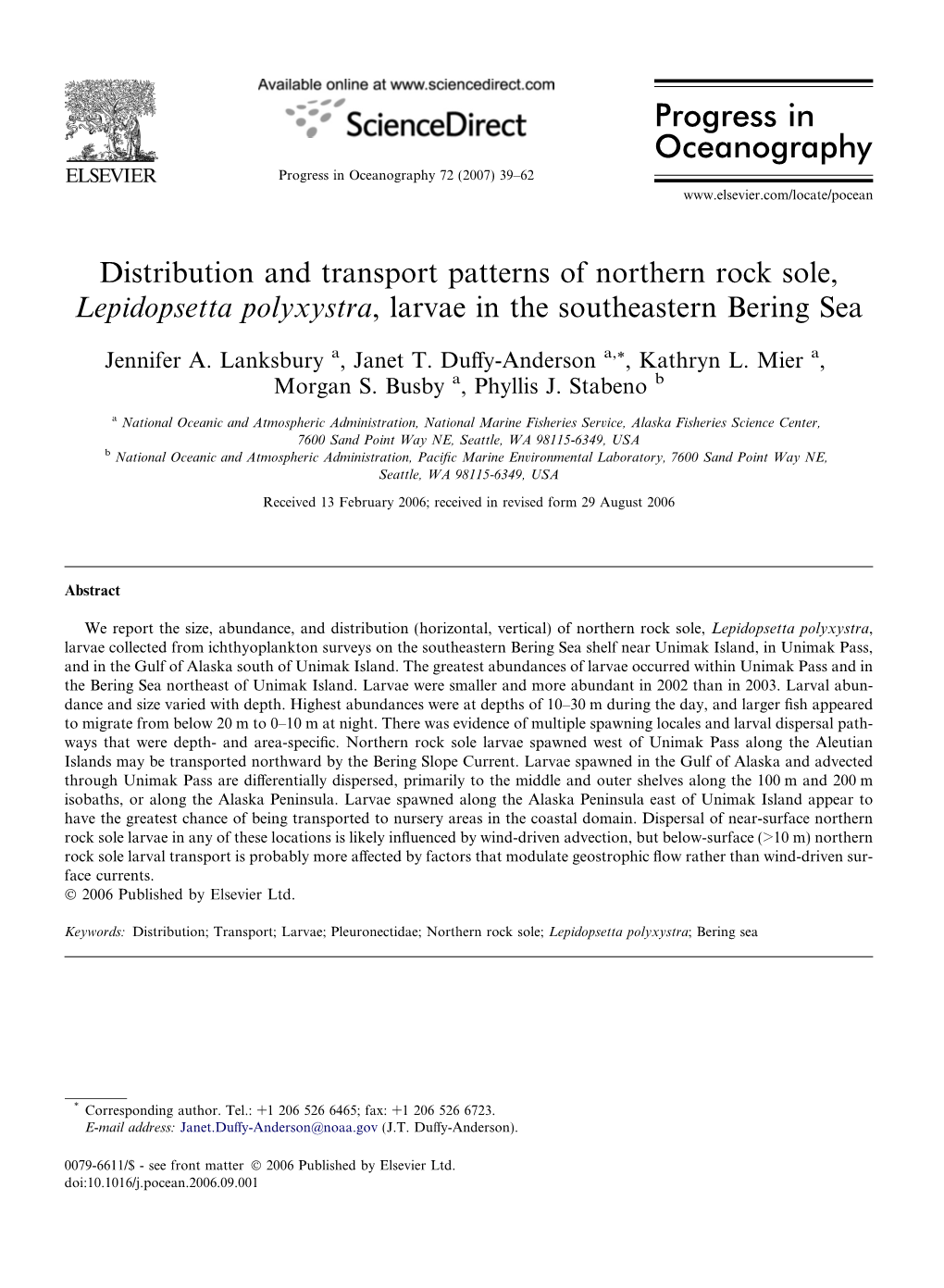
Load more
Recommended publications
-
Fishery Management Plan for Groundfish of the Bering Sea and Aleutian Islands Management Area APPENDICES
FMP for Groundfish of the BSAI Management Area Fishery Management Plan for Groundfish of the Bering Sea and Aleutian Islands Management Area APPENDICES Appendix A History of the Fishery Management Plan ...................................................................... A-1 A.1 Amendments to the FMP ......................................................................................................... A-1 Appendix B Geographical Coordinates of Areas Described in the Fishery Management Plan ..... B-1 B.1 Management Area, Subareas, and Districts ............................................................................. B-1 B.2 Closed Areas ............................................................................................................................ B-2 B.3 PSC Limitation Zones ........................................................................................................... B-18 Appendix C Summary of the American Fisheries Act and Subtitle II ............................................. C-1 C.1 Summary of the American Fisheries Act (AFA) Management Measures ............................... C-1 C.2 Summary of Amendments to AFA in the Coast Guard Authorization Act of 2010 ................ C-2 C.3 American Fisheries Act: Subtitle II Bering Sea Pollock Fishery ............................................ C-4 Appendix D Life History Features and Habitat Requirements of Fishery Management Plan SpeciesD-1 D.1 Walleye pollock (Theragra calcogramma) ............................................................................ -
Ÿþm Icrosoft W
MOODY MARINE LTD Authors: Joe Powers, Geoff Tingley, Susan Hanna, Paul Knapman Public Comment Draft Report for THE GULF OF ALASKA NORTHERN ROCK SOLE TRAWL FISHERY Client: Best Use Coalition Certification Body: Client Contact: Moody Marine Ltd John Gauvin Moody International Certification Best Use Coalition 28 Fleming Drive c/o Groundfish Forum Halifax 4241 21st Ave West Nova Scotia Suite 200 Canada Seattle B3P 1A9 Washington, 98199 Tel: +1 902 489 5581 +1 206 213 5270 FN 82050 Northern Rock Sole GOA V3 January 2010 Page 1 CONTENTS SUMMARY .......................................................................................................................................................4 1. INTRODUCTION ...................................................................................................................................6 1.1 THE FISHERY PROPOSED FOR CERTIFICATION .........................................................................................6 1.2 REPORT STRUCTURE AND ASSESSMENT PROCESS .................................................................................7 1.3 INFORMATION SOURCES USED................................................................................................................8 2 GLOSSARY OF ACRONYMS, ABBREVIATIONS AND SOME DEFINITIONS USED IN THE REPORT...................................................................................................................................13 3 BACKGROUND TO THE FISHERY .................................................................................................15 -
2017 Gulf of Alaska Bottom Trawl Survey
NOAA Technical Memorandum NMFS-AFSC-374 doi:10.7289/V5/TM-AFSC-374 Data Report: 2017 Gulf of Alaska Bottom Trawl Survey P. G. von Szalay and N. W. Raring U.S. DEPARTMENT OF COMMERCE National Oceanic and Atmospheric Administration National Marine Fisheries Service Alaska Fisheries Science Center March 2018 NOAA Technical Memorandum NMFS The National Marine Fisheries Service's Alaska Fisheries Science Center uses the NOAA Technical Memorandum series to issue informal scientific and technical publications when complete formal review and editorial processing are not appropriate or feasible. Documents within this series reflect sound professional work and may be referenced in the formal scientific and technical literature. The NMFS-AFSC Technical Memorandum series of the Alaska Fisheries Science Center continues the NMFS-F/NWC series established in 1970 by the Northwest Fisheries Center. The NMFS-NWFSC series is currently used by the Northwest Fisheries Science Center. This document should be cited as follows: von Szalay, P. G., and N. W. Raring. 2018. Data Report: 2017 Gulf of Alaska bottom trawl survey. U.S. Dep. Commer., NOAA Tech. Memo. NMFS-AFSC-374, 260 p. Document available: http://www.afsc.noaa.gov/Publications/AFSC-TM/NOAA-TM-AFSC-374.pdf Reference in this document to trade names does not imply endorsement by the National Marine Fisheries Service, NOAA. NOAA Technical Memorandum NMFS-AFSC-374 doi:10.7289/V5/TM-AFSC-374 Data Report: 2017 Gulf of Alaska Bottom Trawl Survey P. G. von Szalay and N. W. Raring Resource Assessment and Conservation Engineering Division Alaska Fisheries Science Center National Marine Fisheries Service National Oceanic and Atmospheric Administration 7600 Sand Point Way N.E. -
Stock Status Table
National Marine Fisheries Service - 2020 Status of U.S. Fisheries Table A. Summary of Stock Status for FSSI Stocks Overfishing? Overfished? (Is Fishing Management Rebuilding (Is Biomass Approaching Jurisdiction FMP Stock Mortality Action Program B/B Points below Overfished MSY above Required Progress Threshold?) Threshold?) Consolidated Atlantic Highly Atlantic sharpnose shark - Atlantic HMS No No No NA NA 2.08 4 Migratory Species Atlantic Consolidated Atlantic Highly Atlantic sharpnose shark - Atlantic HMS No No No NA NA 1.02 4 Migratory Species Gulf of Mexico Reduce Consolidated Atlantic Highly Mortality, Year 8 of 30- Atlantic HMS Blacknose shark - Atlantic Yes Yes NA 0.43-0.64 1 Migratory Species Continue year plan Rebuilding Consolidated Atlantic Highly not Atlantic HMS Blacktip shark - Atlantic Unknown Unknown Unknown NA NA 0 Migratory Species estimated Consolidated Atlantic Highly Blacktip shark - Gulf of Atlantic HMS No No No NA NA 2.62 4 Migratory Species Mexico Consolidated Atlantic Highly Finetooth shark - Atlantic Atlantic HMS No No No NA NA 1.30 4 Migratory Species and Gulf of Mexico Consolidated Atlantic Highly Great hammerhead - Atlantic not Atlantic HMS Unknown Unknown Unknown NA NA 0 Migratory Species and Gulf of Mexico estimated Consolidated Atlantic Highly Lemon shark - Atlantic and not Atlantic HMS Unknown Unknown Unknown NA NA 0 Migratory Species Gulf of Mexico estimated Consolidated Atlantic Highly Sandbar shark - Atlantic and Continue Year 16 of 66- Atlantic HMS No Yes NA 0.77 2 Migratory Species Gulf of Mexico -
Fishes-Of-The-Salish-Sea-Pp18.Pdf
NOAA Professional Paper NMFS 18 Fishes of the Salish Sea: a compilation and distributional analysis Theodore W. Pietsch James W. Orr September 2015 U.S. Department of Commerce NOAA Professional Penny Pritzker Secretary of Commerce Papers NMFS National Oceanic and Atmospheric Administration Kathryn D. Sullivan Scientifi c Editor Administrator Richard Langton National Marine Fisheries Service National Marine Northeast Fisheries Science Center Fisheries Service Maine Field Station Eileen Sobeck 17 Godfrey Drive, Suite 1 Assistant Administrator Orono, Maine 04473 for Fisheries Associate Editor Kathryn Dennis National Marine Fisheries Service Offi ce of Science and Technology Fisheries Research and Monitoring Division 1845 Wasp Blvd., Bldg. 178 Honolulu, Hawaii 96818 Managing Editor Shelley Arenas National Marine Fisheries Service Scientifi c Publications Offi ce 7600 Sand Point Way NE Seattle, Washington 98115 Editorial Committee Ann C. Matarese National Marine Fisheries Service James W. Orr National Marine Fisheries Service - The NOAA Professional Paper NMFS (ISSN 1931-4590) series is published by the Scientifi c Publications Offi ce, National Marine Fisheries Service, The NOAA Professional Paper NMFS series carries peer-reviewed, lengthy original NOAA, 7600 Sand Point Way NE, research reports, taxonomic keys, species synopses, fl ora and fauna studies, and data- Seattle, WA 98115. intensive reports on investigations in fi shery science, engineering, and economics. The Secretary of Commerce has Copies of the NOAA Professional Paper NMFS series are available free in limited determined that the publication of numbers to government agencies, both federal and state. They are also available in this series is necessary in the transac- exchange for other scientifi c and technical publications in the marine sciences. -
Comparisons of Diet and Nutritional Conditions in Pseudopleuronectes Herzensteini Juveniles Between Two Nursery Title Grounds Off Northern Hokkaido, Japan
Comparisons of diet and nutritional conditions in Pseudopleuronectes herzensteini juveniles between two nursery Title grounds off northern Hokkaido, Japan Author(s) Kobayashi, Yuki; Takatsu, Tetsuya; Yamaguchi, Hiroshi; Joh, Mikimasa Fisheries science, 81(3), 463-472 Citation https://doi.org/10.1007/s12562-015-0860-0 Issue Date 2015-05 Doc URL http://hdl.handle.net/2115/59346 Rights The final publication is available at www.springerlink.com Type article (author version) File Information Fish Sci 81 Takatsu.pdf Instructions for use Hokkaido University Collection of Scholarly and Academic Papers : HUSCAP 1 Comparisons of diet and nutritional condition in Pseudopleuronectes herzensteini 2 juveniles between two nursery grounds off northern Hokkaido, Japan 3 Yuki Kobayashi, Tetsuya Takatsu, Hiroshi Yamaguchi, Mikimasa Joh 4 5 Y. Kobayashi · T. Takatsu (corresponding author) 6 Graduate School of Fisheries Sciences and Faculty of Fisheries Sciences, Hokkaido 7 University, Hakodate, Hokkaido 041-8611, Japan 8 e-mail: [email protected] [email protected] Tel/Fax 9 +81-138-40-8822 10 11 H. Yamaguchi 12 Wakkanai Fisheries Research Institute, Hokkaido Research Organization, Suehiro 13 4-5-15, Wakkanai, Hokkaido 097-0001, Japan 14 e-mail: [email protected] 15 16 M. Joh 17 Abashiri Fisheries Research Institute, Hokkaido Research Organization, Masuura 1-1-1, 18 Abashiri, Hokkaido 099-3119, Japan 19 e-mail: [email protected] 20 21 Present address: 22 M. Joh 23 Mariculture Fisheries Research Institute, Hokkaido Research Organization, Funemi 24 1-156-3, Muroran, Hokkaido 051-0013, Japan 1 1 Abstract 2 To characterise food-habits differences in Pseudopleuronectes herzensteini juveniles, 3 we compared diets, prey diversity and nutritional states between two groups i.e., one in 4 the Sea of Japan and the other in the Sea of Okhotsk around northern Hokkaido, Japan. -
Biological Characterization: an Overview of Bristol, Nushagak, and Kvichak Bays; Essential Fish Habitat, Processes, and Species Assemblages
AN ASSESSMENT OF POTENTIAL MINING IMPACTS ON SALMON ECOSYSTEMS OF BRISTOL BAY, ALASKA VOLUME 3—APPENDICES E-J Appendix F: Biological Characterization: Bristol Bay Marine Estuarine Processes, Fish and Marine Mammal Assemblages Biological Characterization: An Overview of Bristol, Nushagak, and Kvichak Bays; Essential Fish Habitat, Processes, and Species Assemblages December 2013 Prepared by National Marine Fisheries Service, Alaska Region National Marine Fisheries Service, Alaska Region ii PREFACE The Bristol Bay watershed supports abundant populations of all five species of Pacific salmon found in North America (sockeye, Chinook, chum, coho, and pink), including nearly half of the world’s commercial sockeye salmon harvest. This abundance results from and, in turn, contributes to the healthy condition of the watershed’s habitat. In addition to these fisheries resources, the Bristol Bay region has been found to contain extensive deposits of low-grade porphyry copper, gold, and molybdenum in the Nushagak and Kvichak River watersheds. Exploration of these deposits suggests that the region has the potential to become one of the largest mining developments in the world. The potential environmental impacts from large-scale mining activities in these salmon habitats raise concerns about the sustainability of these fisheries for Alaska Natives who maintain a salmon-based culture and a subsistence lifestyle. Nine federally recognized tribes in Bristol Bay along with other tribal organizations, groups, and individuals have petitioned the U.S. Environmental -
Gulf of Alaska Groundfish
Fishery Management Plan for Groundfish of the Gulf of Alaska APPENDICES Appendix A History of the Fishery Management Plan ................................................................ A-1 A.1 Amendments to the FMP ................................................................................................... A-1 Appendix B Geographical Coordinates of Areas Described in the Fishery Management Plan B- 1 B.1 Management Area, Regulatory Areas and Districts .......................................................... B-1 B.1.1 Management Area .............................................................................................. B-1 B.1.2 Regulatory Areas ............................................................................................... B-1 B.1.3 Districts .............................................................................................................. B-2 B.2 Closed Areas ...................................................................................................................... B-2 B.2.1 Sitka Pinnacles Marine Reserve ........................................................................ B-2 B.2.2 Marmot Bay Tanner Crab Protection Area ........................................................ B-2 B.2.3 King Crab Closures around Kodiak Island ........................................................ B-4 B.2.4 Cook Inlet non-Pelagic Trawl Closure Area ...................................................... B-6 B.2.5 Alaska Seamount Habitat Protection Area (ASHPA) ....................................... -
BSAI Northern Rock Sole Assessment Raised an Important Issue About Using Localized Gear Performance Studies (E.G
Chapter 8 Assessment of the Northern Rock Sole stock in the Bering Sea and Aleutian Islands Thomas K. Wilderbuer and Daniel G. Nichol Executive Summary The following changes have been made to this assessment relative to the last full assessment in November 2015: Summary of changes to the assessment input 1) 2015 fishery age composition. 2) 2015 survey age composition. 3) 2016 trawl survey biomass point estimates and standard errors. 4) Estimate of catch (t) for 2016. 5) Estimate of retained and discarded portions of the 2016 catch. Summary of Results Models 1 is the preferred model evaluated in this assessment. Models 2-7 represent Model runs made to examine alternate states of nature for contrast to the primary models results. Northern rock sole continue to be moderately exploited and the female spawning biomass is in a slow decline but well-above BMSY. Model 1 As estimated or As estimated or specified last year for: recommended this year for: Quantity 2016 2017 2017 2018 M (natural mortality rate) 0.15 0.15 0.15 0.15 Tier 1a 1a 1a 1a Projected total (age 6+) 1,085,200 977,200 1,000,600 923,200 Female spawning biomass (t) 584,400 522,600 539,500 472,200 Projected B0 682,800 918,500 BMSY 257,000 257,000 257,000 257,000 FOFL 0.152 0.152 0.160 0.160 maxFABC 0.148 0.148 0.155 0.155 FABC 0.148 0.148 0.155 0.155 OFL (t) 165,900 149,400 159,700 147,300 maxABC (t) 161,000 145,000 155,100 143,100 ABC (t) 161,000 145,000 155,100 143,100 Status As determined last year for: As determined this year 2014 2015 2015 2016 Overfishing No No No No Overfished No No No No Approaching overfished No No No No Model 1 Responses to SSC and Plan Team Comments to Assessments in General The Plan Team discussion relative to its choice of a preferred model for the BSAI northern rock sole assessment raised an important issue about using localized gear performance studies (e.g. -
Alaska Flatfish RFM Certificate
RESPONSIBLE FISHERIES MANAGEMENT CERTIFICATE Certificate no.: Initial certification date: Valid until: 10000445829-MSC-ANSI-USA 18 November, 2016 03 December, 2024 This is to certify that the Fishery operations of Alaska Flatfish Fishery Alaska Seafood Cooperative, 4241 21st Ave W. Suite 302, Seattle, WA 98199-1250, USA has been found to conform to the requirements of: Alaska Responsible Fisheries Management (RFM) Certification Scheme Version 1.3 as derived from 1995 FAO Code of Conduct for Responsible Fisheries and 2005/2009 FAO Guidelines for Ecolabelling of Fish and Fishery Products from Marine Capture Fisheries. This certificate is valid for the following fishery: Fishery name: Alaska Flatfish Fishery Species (latin and common name): Alaska plaice (Pleuronectes quadrituberculatus), Arrowtooth flounder (Atheresthes stomias), Flathead sole (Hippoglossoides elassodon), Greenland turbot (Reinhardtius hippoglossoides), Kamchatka flounder (Atheresthes evermanni), Northern rock sole (Lepidopsetta polyxstra), Yellowfin sole (Limanda aspera), Southern rock sole (Lepidopsetta bilineatus), Rex sole (Glyptocephalus zachirus) Geographical area: Gulf of Alaska (GOA) and Bering Sea and Aleutian Islands (BSAI) within Alaska jurisdiction (200 nautical miles EEZ) Method of capture: Bottom trawl Stock: BSAI – Alaska plaice, Arrowtooth flounder, Flathead sole, Greenland turbot, Kamchatka flounder, Northern rock sole, Yellowfin sole GOA – Arrowtooth flounder, Flathead sole, Northern rock sole, Southern rock sole, Rex sole Management: National Marine Fisheries Service; North Pacific Fishery Management Council; National Oceanic and Atmospheric Administration Client group: Alaska Seafood Cooperative The certified fishery has the right to claim the fishery is a “Well Managed and Responsible Fishery”, in accordance with the RFM’s FAO based criteria for responsible fishing. Further claims made about the fishery shall be in accordance with the rules established by Certified Seafood Collaborative. -
Alaska Flatfish Commercial Fisheries (200 Mile EEZ) Facilitated by The
FAO-Based Responsible Fisheries Management AK Flatfish 1st Surveillance Report FAO-BASED RESPONSIBLE FISHERY MANAGEMENT CERTIFICATION SURVEILLANCE REPORT For The Alaska Flatfish Commercial Fisheries (200 mile EEZ) Facilitated By the Alaska Seafood Marketing Institute (ASMI) Assessors: Vito Ciccia Romito; Lead Assessor Jeff Fargo; Assessor Geraldine Criquet, PhD; Assessor Report Code: AK/FLAT/001.1/2014 Published in March 2015 SAI Global/Global Trust Certification Ltd. Head Office, 3rd Floor, Block 3, Quayside Business Park, Mill Street, Dundalk, Co. Louth. T: +353 42 9320912 F: +353 42 9386864 web: www.GTCert.com Form 11b Issue 1 Dec 2011 Page 1 of 155 FAO-Based Responsible Fisheries Management AK Flatfish 1st Surveillance Report Contents I. Summary and Recommendations ................................................................................................ 3 II. Assessment Team Details ............................................................................................................. 4 III. Acronyms ...................................................................................................................................... 5 1. Introduction .................................................................................................................................. 7 1.1. Recommendation of the Assessment Team ................................................................................. 8 2. Fishery Applicant Details ............................................................................................................. -
Walleye Pollock Russia Midwater Trawls
Walleye pollock Gadus chalcogrammus ©Scandinavian Fishing Yearbook/www.scandposters.com Russia Midwater trawls, Danish seines December 4, 2017 Seafood Watch Consulting Researcher Disclaimer Seafood Watch® strives to have all Seafood Reports reviewed for accuracy and completeness by external scientists with expertise in ecology, fisheries science and aquaculture. Scientific review, however, does not constitute an endorsement of the Seafood Watch program or its recommendations on the part of the reviewing scientists. Seafood Watch is solely responsible for the conclusions reached in this report. Seafood Watch Standard used in this assessment: Standard for Fisheries vF3 Table of Contents About. Seafood. .Watch . 3. Guiding. .Principles . 4. Summary. 5. Final. Seafood. .Recommendations . 7. Introduction. 9. Assessment. 15. Criterion. 1:. .Impacts . on. the. Species. Under. Assessment. .15 . Criterion. 2:. .Impacts . on. Other. Species. .24 . Criterion. 3:. .Management . Effectiveness. .39 . Criterion. 4:. .Impacts . on. the. Habitat. .and . Ecosystem. .48 . Acknowledgements. 53. References. 54. Appendix. A:. Extra. .By . Catch. .Species . 59. 2 About Seafood Watch Monterey Bay Aquarium’s Seafood Watch program evaluates the ecological sustainability of wild-caught and farmed seafood commonly found in the United States marketplace. Seafood Watch defines sustainable seafood as originating from sources, whether wild-caught or farmed, which can maintain or increase production in the long-term without jeopardizing the structure or function of affected ecosystems. Seafood Watch makes its science-based recommendations available to the public in the form of regional pocket guides that can be downloaded from www.seafoodwatch.org. The program’s goals are to raise awareness of important ocean conservation issues and empower seafood consumers and businesses to make choices for healthy oceans.