A Comparison of Exoplanet Spectroscopic Retrieval Tools
Total Page:16
File Type:pdf, Size:1020Kb
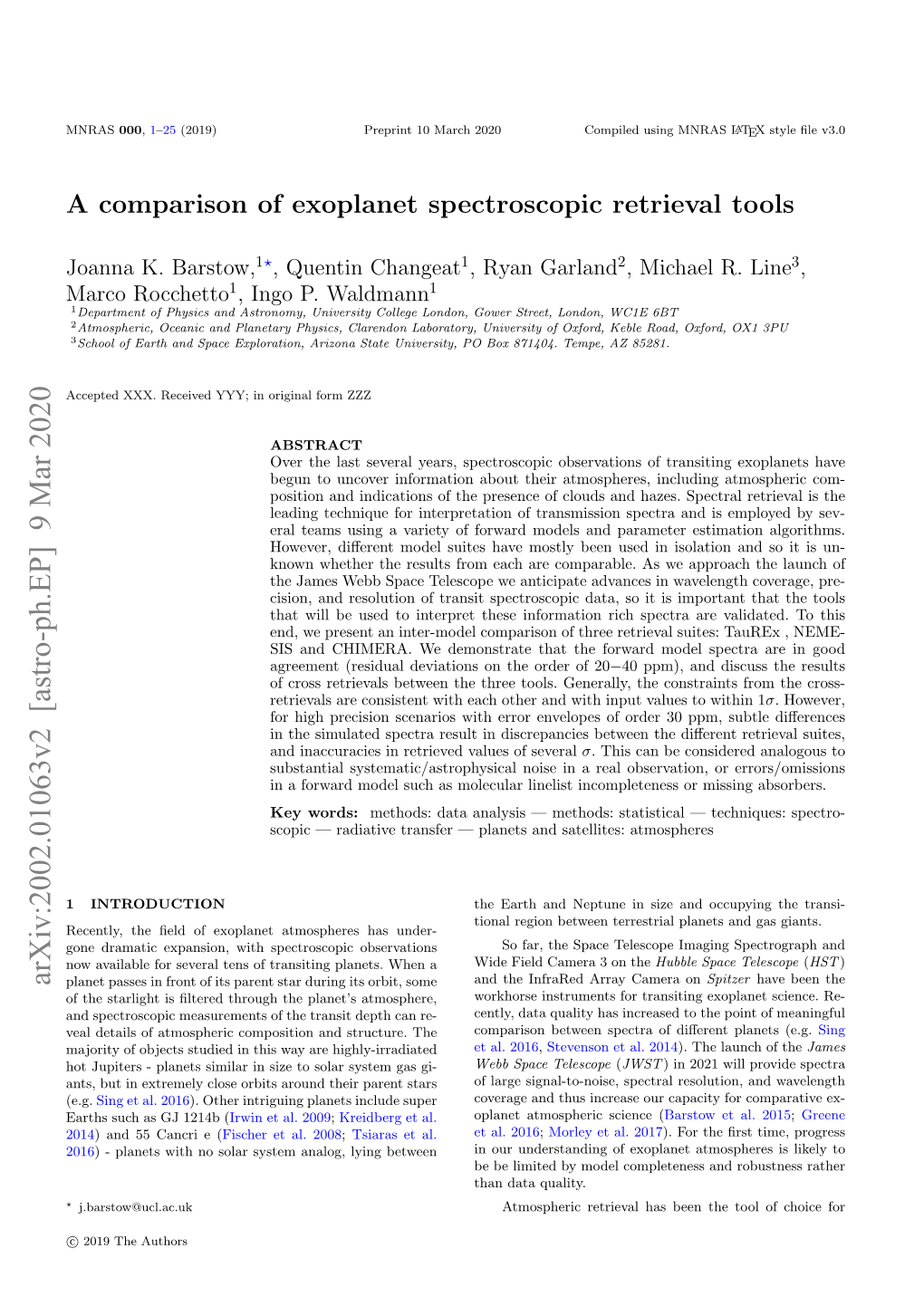
Load more
Recommended publications
-
A Comparison of Exoplanet Retrieval Tools
EPSC Abstracts Vol. 12, EPSC2018-467, 2018 European Planetary Science Congress 2018 EEuropeaPn PlanetarSy Science CCongress c Author(s) 2018 A comparison of exoplanet retrieval tools Joanna K. Barstow (1), Ryan Garland (2), Michael R. Line (3), Marco Rocchetto (1), and Ingo P. Waldmann (1) (1) Department of Physics and Astronomy, University College London, UK (2) Atmospheric, Oceanic and Planetary Physics, University of Oxford, UK (3) School of Earth and Space Exploration, Arizona State University, Arizona, USA ([email protected]) Abstract nique has been used extensively (e.g. [5, 6, 1, 7]) and is acknowledged to be an efficient and reliable method In recent years, spectroscopic observations of tran- for constraining exoplanet atmospheres from transmis- siting exoplanets have begun to uncover information sion and eclipse spectra. Whilst all retrieval codes fol- about their atmospheres including atmospheric struc- low the same basic structure, there is substantial vari- ture and composition, and indications of the presence ation in both the forward model set up and the method of clouds. Spectral retrieval is the leading technique used to solve the inverse problem, leading to the pos- for interpretation of exoplanet transmission spectra. sibility that two different retrieval codes may provide Whilst several atmospheric models and retrieval algo- vastly different solutions to the same dataset (e.g. the rithms have been successfully employed, as yet the dif- four analysis of WASP-63b presented by [3]). ferent model suites have mostly been used in isolation To test the robustness of the retrieval approach to and so it is unknown whether results from each are this sort of issue we here present a comparison of three comparable. -
Survivability of Planetary Systems in Young and Dense Star Clusters the Free-floating Stars in the Cluster
Astronomy & Astrophysics manuscript no. rogue_planets c ESO 2019 March 12, 2019 Survivability of planetary systems in young and dense star clusters A. van Elteren1, S. Portegies Zwart⋆1, I. Pelupessy2, Maxwell X. Cai1, and S.L.W. McMillan3 1 Leiden Observatory, Leiden University, NL-2300RA Leiden, The Netherlands 2 The Netherlands eScience Center, The Netherlands 3 Drexel University, Department of Physics, Philadelphia, PA 19104, USA Accepted XXX. Received YYY; in original form ZZZ ABSTRACT Aims. We perform a simulation using the Astrophysical Multipurpose Software Environment of the Orion Trapezium star cluster in which the evolution of the stars and the dynamics of planetary systems are taken into account. Methods. The initial conditions from earlier simulations were selected in which the size and mass distributions of the observed circumstellar disks in this cluster are satisfactorily reproduced. Four, five, or size planets per star were introduced in orbit around the 500 solar-like stars with a maximum orbital separation of 400 au. Results. Our study focuses on the production of free-floating planets. A total of 357 become unbound from a total of 2522 planets in the initial conditions of the simulation. Of these, 281 leave the cluster within the crossing timescale of the star cluster; the others remain bound to the cluster as free-floating intra-cluster planets. Five of these free-floating intra-cluster planets are captured at a later time by another star. Conclusions. The two main mechanisms by which planets are lost from their host star, ejection upon a strong encounter with another star or internal planetary scattering, drive the evaporation independent of planet mass of orbital separation at birth. -
Brendan Krueger Phy 688, Spring 2009 May 6Th, 2009 Development of the Theory
Brendan Krueger Phy 688, Spring 2009 May 6th, 2009 Development of the theory . Alvarez hypothesis . Periodicity of extinctions . Proposal of a solar companion Orbit of Nemesis . Proposed orbit . Current location . Stability Detection of Nemesis . Why we haven’t seen it . Pan‐STARRS . LSST Alternate theory . Oscillation through the galactic plane Nemesis, Brendan Krueger 2 The Alvarez hypothesis Periodicity of extinctions Proposal of a solar companion Nemesis, Brendan Krueger 3 Alvarez !"#$%&# (1980) Depletion of platinum metals (Ru, Rh, Pd, Os, Ir, Pt) on Earth Increased iridium coincident with Cretaceous‐ Tertiary extinction (K‐T Event) Massive asteroid impact . Diameter: 10 ± 4 km . Abundances suggest impactor origin is solar, but extraterrestrial . Spread dust throughout atmosphere ▪ Extinction (block sunlight, etc.) ▪ Iridium‐rich layer in geologic record Nemesis, Brendan Krueger 4 Alvarez !"#$%&# (1980) 4C6EE/-shoe-lube: Artist&s conception o the -T Eent (thus saith the Internets and phone calls to Mexico aren&t cheap) “Maybe an Asteroid '()*+"$Kill the Dinosaurs”, Jeffrey Kluger, TIME, 27 April, 2009 Nemesis, Brendan Krueger 5 Raup & Sepkoski (1984) Various periodic analysis techniques reveal spikes in the extinction record around every 30 Myr Best fit period evidenced a cycle of 26Myr Period‐folding the data displays a relatively sharp peak . Discrete extinction events Nemesis, Brendan Krueger 6 Raup & Sepkoski (1984) Various periodic analysis techniques reveal spikes in the extinction record around every 30 Myr Best fit period evidenced a cycle of 26Myr Period‐folding the data displays a relatively sharp peak . Discrete extinction events Nemesis, Brendan Krueger 7 Raup & Sepkoski (1984) Two events known to match impact events (K‐T Event and Late Eocene extinction) Length of cycle suggests extraterrestrial source Uncertainty in geologic time Nemesis, Brendan Krueger 8 Whitmire & Jackson (1984); Davis, Hut, Muller (1984) Propose a low‐mass solar companion . -
Perturbing Events in the Galaxy
_3~______________________________________ BOOKREVIEWS _____________________N_A_TU_R_E_V_O_L_._32_8_30_1_U_L_Y_19_87 Sun orbits around in the Galaxy, are too investigation of the precision of the strati Perturbing events small to affect significantly the number of graphical dating have now been com comets perturbed towards the Earth by pleted. There are well-established and in the Galaxy interstellar clouds or by the variable gal reliably dated impact events and bio Gerry Gilmore actic tide. So could a solar companion - logical extinctions at - 35 Myr and - 65 planet or star - cause a comet shower of Myr ago, so that cometary impacts do sufficient intensity to guarantee a large indeed seem to cause mass extinctions. The Galaxy and the Solar System. Edited impact on the Earth? Given the low prob But earlier events are less well-dated, and by Roman Smoluchowski, John N. ability of such an impact, several billion the claimed periodicity, on which the Bahcall and Mildred S. Matthews. Uni comets would be required. One or more validity of the companion-star model versity of Arizona Press: 1986. Pp.483. per day would cross the Earth's orbit, relied, is not statistically significant. In $29.95. providing an entertaining spectacle at consequence of this, the exhaustive and least. repetitive discussion of the relevant argu FEW, if any, dinosaurs will read this A tenth planet seems unable to account ments in this volume becomes of mostly review. It is also well established that the for this. Reasonably detailed dynamical historical interest. While it makes fascina biological extinction at the Cretaceous - modelling shows that the comet showers it ting reading, the book is more appro Tertiary boundary about 65 Myr ago co caused would be too long-lasting and of priate for a long journey than as a text or incided with a period of increased impact too low contrast to the background rate. -
Nemesis, Tyche, Planet Nine Hypotheses. I. Can We Detect the Bodies Using Gravitational Lensing?
Publications of the Astronomical Society of Australia (PASA), Vol. 33, e033, 13 pages (2016). C Astronomical Society of Australia 2016; published by Cambridge University Press. doi:10.1017/pasa.2016.28 Nemesis, Tyche, Planet Nine Hypotheses. I. Can We Detect the Bodies Using Gravitational Lensing? J. P. Philippov1,3 and M. I. Chobanu2 1Samara State University, Academica Pavlova st 1, 443011, Samara, Russian Federation 2Samara International Aerospace Lyceum, Lukacheva st 45, 443086, Samara, Russian Federation 3Email: [email protected] (Received December 21, 2015; Accepted July 18, 2016) Abstract In this paper, the hypothesis of the existence of a massive dark body (Nemesis, Tyche, Planet Nine, or any other trans- Plutonian planet) at the Solar system periphery is analysed. Basic physical properties and orbital characteristics of such massive bodies are considered. The problem of the definition of a scattering angle of a photon in the gravitational field of a spherical lens is studied. It is shown that, the required value of the scattering angle can be measured for the cases of Nemesis and Tyche. The formation of gravitational lensing images is studied here for a point mass event. It is demonstrated that in most cases of the close rapprochement of a source and the lens (for Nemesis and Tyche), it is possible to resolve two images. The possibility of resolving these images is one of the main arguments favouring the gravitational lensing method as its efficiency in searching for dark massive objects at the edge of the Solar System is higher than the one corresponding to other methods such as stellar occultation. -
Is There Planetx/Nemesis?
EPSC Abstracts Vol. 5, EPSC2010-614, 2010 European Planetary Science Congress 2010 c Author(s) 2010 Is there PlanetX/Nemesis? L. Iorio F.R.A.S. Viale Unità di Italia 68, 70125, Bari (BA) Italy ([email protected]) Abstract us, its orbital period would amount to 1-10 Myr, con- trary to the 26 Myr periodicity in extinction rates on We use the precessions of the perihelia of some plan- the Earth over the last 250 Myr which motivated the ets of our Sun to preliminarily constrain the minimum Nemesis proposal. Moreover, our Sun-sized body X distance at which a putative distant object may exist in would not penetrate the Oort cloud which is believed the remote peripheries of the solar system for different to extend from 50 kau to 150 kau. The tidal parameter of Nemesis would be, instead, 2 4 orders of magni- values of its mass. − tude smaller than the present-day level of accuracy in 26 2 measuring it (10− s− ). On the other hand, if our X had a distance of about 88 kau, as predicted for Neme- 1 Planetary perturbations of a sis, our result for its tidal parameter would imply a mass of 300M . putative X/Nemesis For a particular position of X, i.e. along the direc- We have shown that a putative distant body X, not yet tion of the Galactic Center, our results hold also for the discovered, would induce non-vanishing secular pre- recently proposed form of the External Field Effect in cessions of the longitudes of the perihelion and the the framework of MOND in the sense that it would be node of a known planet P of the solar system. -
Machine Learning in Exoplanet Atmospheric Characterisation Ariel Conference 2020
Machine Learning in Exoplanet Atmospheric Characterisation Ariel Conference 2020 Dr Ingo Waldmann TauREx 3 3 • Built from the ground up as full python stack • 10 - 200 times faster than TauREx 2 • Full NVIDIA and OpenCL GPU support (another 50x faster for JWST or high-res) A PREPRINT -OCTOBER 21, 2019 • Fully tested against TauREx 2 which is benchmarked against NEMESIS, CHIMERA, ARCiS ⌧-REx 2 ⌧-REx 2 ⌧-REx 3 Molecules xsec (s) k-tables (s) xsec (s) • For full installation type: “pip install taurex” 1 7.23 0.45 0.61 • Plugin features and TauREx extensions 2 8.90 0.78 0.74 4 12.42 1.49 0.92 7 19.02 2.63 1.23 • New and fast cross sections 15 263.56 8.21 2.34 • Fully open under BSDTable license 6: A comparison of the forwardAl-Rafaie model et computation al. submitted, time between arXiv: TauREx1912:07759 2 using cross-sections, TauREx 2 using k-tables and TauREx 3 using cross-sections for the same atmospheric parameters but increasing number of molecules https://github.com/ucl-exoplanets/TauREx3 ⌧-REx 2 ⌧-REx 3 R xsec (s) xsec (s) 7000 0.57 0.039 10000 0.85 0.062 15000 1.02 0.092 Table 7: Comparing ⌧-REx 2 and ⌧-REx 3 in the region of HST with different cross-section resolution model as before and test with three different resolutions of cross-sections. The wavelength region considered is 1.1 1.7µm which is the same as the Hubble Space Telescope. ⇡ − Table 7.1 highlights how cross-section resolution influcences the computational time of TauRex3 reaching around 10x performance gain which should give significantly shorter retrieval times. -
History, Expansionism, and Guardianship in Isaac Asimov’S Science Fiction
The Cowboy PoliTiCs of an enlighTened fuTure: History, Expansionism, and Guardianship in Isaac Asimov’s Science Fiction JARI KÄKELÄ UNIVERSITY OF HELSINKI FACULTY OF ARTS The Cowboy PoliTiCs of an enlighTened fuTure: History, Expansionism, and Guardianship in Isaac Asimov’s Science Fiction Jari KÄKelÄ Department of Modern Languages University of Helsinki © Jari Käkelä 2016 Layout and cover design by Jari Käkelä Cover background image: www.pixabay.com, public domain; foreground diagram collage by Jari Käkelä. ISBN 978-951-51-2404-3 (paperback) ISBN 978-951-51-2405-0 (PDF) Helsinki, 2016 ABSTRACT Isaac Asimov (1920–1992) was one of the central writers of the formative period of importance of the genre. This dissertation examines the themes of history, frontier expansionism, and guardianship in Asimov’s key works, the Robot and Foundation Robot and Foundation as serials in the 1940s and 1950s Astounding Science-Fiction publishing context is crucial in order to understand Asimov’s impact on the genre. Thus, this dissertation combines the contextual examination of Asimov’s main themes with a discussion of the views of the Astounding history. construct a sustainable future becomes the pivotal theme, both on the level of narration and on the level of characters that turn their knowledge of history into action. This will decline if stagnation is not reversed by frontier expansion. The pervasive frontier the intellectual frontier of the future. Finally, the historical and frontier aspects in Asimov’s series point toward the notion of guardianship and the aspiration to apply the understanding of both history and science to engineer a more peaceful, yet non- stagnant future. -
Exoplanet Research at the University of Oxford
Exoplanet Research at the University of Oxford Patrick Irwin Oxford Astro/AOPP Who’s who ! Astrophysics ! Transit modelling and Stellar Variability – Suzanne Aigrain ! Hannu Parviainen, Ben Pope, Ed Gillen, Ruth Angus, Vinesh Rajpaul ! Planet formation modelling – Caroline Terquem ! Telescope Instrumentation – Niranjan Thatte ! Matthias Tecza, Fraser Clarke ! Atmospheric, Oceanic and Planetary Physics ! Radiative Transfer and Retrievals – Patrick Irwin ! Exoplanet atmospheric characterisation (transit spectroscopy) and clouds – Jo Barstow, Leigh Fletcher ! Brown dwarf atmospheric characterisation – Ryan Garland, Jo Barstow ! Instrumentation – Neil Bowles, Simon Calcutt ! EChO, Ariel ! GCM modelling – Peter Read Kepler – 2 at Oxford › Group: - Oxford: Suzanne Aigrain, Steve Roberts, Hannu Parviainen, Ed Gillen, Benjamin Pope - Cambridge: Simon Hodgkin, Mike Irwin, Jonathan Irwin, Jim Lewis › Kepler-2 is the two-wheel successor to the Kepler mission › Consists of a sequence of ~ 80 day ecliptic plane campaigns › Poor pointing stability requires careful correction of systematics in the photometry › Exciting cluster science - ecliptic pointings take in Pleiades, Hyades, M35, ρ Oph and more! 7 Kepler – 2 Lightcurves › Left to right: - Raw light curve - Roll angle – dependent Gaussian Process fit - New light curve with roll angle-related systematics subtracted - Note at the bottom: large amplitude signals from stellar variability distinguished from pointing jitter and unaffected by calibration 8 Young eclipsing binaries in NGC 2264 (~3 Myr) -
On the Possibility of Binary Companion of the Sun Perspective
Prespacetime Journal| February 2020 | Volume 11 | Issue 1 | pp. xxx-xxx 100 Christianto, V., & Smarandache, F., On the Possibility of Binary Companion of the Sun Perspective On the Possibility of Binary Companion of the Sun Victor Christianto1* & Florentin Smarandache2 1Malang Institute of Agriculture (IPM), Malang, Indonesia 2Dept. of Math. Sci., Univ. of New Mexico, Gallup, USA Abstract In this article, we present some new arguments on the theoretical dwarf star thought to be a companion to our Sun, known as the Nemesis, which is postulated to explain a perceived cycle of mass extinctions in Earth's history. Some speculated that such a star could affect the orbit of objects in the far outer solar system, sending them on a collision course with Earth. While recent astronomical surveys failed to find any evidence that such a star exists, we outline in this article some theoretical findings including our own serendipity finding, suggesting that such a dwarf star companion of the Sun remains a plausibility. We also discuss possible theoretical framework to advance further this line of thought. Keywords: Planet nine, dwarf star, binary star formation, solar system. Introduction The existence of a dwarf star as binary companion of the Sun has been debated for long time, although its existence has not been found yet. Some argues that no evidence at all supports such a dwarf star companion, sometimes dubbed as Nemesis. Nemesis is a theoretical dwarf star thought to be a companion to our sun. The theory was postulated to explain a perceived cycle of mass extinctions in Earth's history. -
Measurements of Lunar Impacts ... and Implications for the Nemesis Theory
Geological Society of America Special Paper 356 2002 Measurement of the lunar impact record for the past 3.5 b.y. and implications for the Nemesis theory Richard A. Muller* Department of Physics and Lawrence Berkeley Laboratory, 50-5032 LBL, University of California, Berkeley, California 94720, USA ABSTRACT Measurements of the ages of 155 lunar spherules from the Apollo 14 site suggest that the solar system impact rate over the past 3.5 b.y. first gradually declined, and then increased starting at 0.4 Ga, back to the level it had been 3 Ga. A possible explanation is offered in terms of the Nemesis theory, which postulated a solar com- panion star. A sudden change in the orbit of that star at 0.4 Ga transformed a circular orbit (which does not trigger comet showers) into an eccentric orbit (which does). The Nemesis theory is speculative but viable; contrary to prior assertions, the orbit is sufficiently stable to account for the data. INTRODUCTION theory (Davis et al., 1984), which predicts that the impact rate will have a large component from periodic comet showers, A new approach to estimating past impact rates has been events in which multiple impacts occur within short (1–2 m.y.) developed by our group at Berkeley: rather than measuring the time spans. Moreover, the theory predicts that the comet show- ages of identified craters, the ages of small glass droplets called ers will recur whenever the cause (Nemesis, the hypothetical spherules that are produced from unidentified impact craters are companion star to the Sun) reaches perihelion, which is once measured (Muller, 1993). -
Exoplanet Atmospheres with Echo: Spectral Retrievals Using Echosim
Exoplanet atmospheres with EChO: spectral retrievals using EChOSim Joanna K. Barstow1,*, Neil E. Bowles1, Suzanne Aigrain1, Leigh N. Fletcher1, Patrick G. J. Irwin1, Ryan Varley2, Enzo Pascale3 1 Department of Physics, University of Oxford, Oxford, UK 2 Department of Physics, University College London, London, UK 3School of Physics and Astronomy, University of Cardiff, Cardiff, UK *[email protected] We demonstrate the effectiveness of the Exoplanet Characterisation Observatory mission concept for constraining the atmospheric properties of hot and warm gas giants and super Earths. Synthetic primary and secondary transit spectra for a range of planets are passed through EChOSim (Waldmann & Pascale 2014) to obtain the expected level of noise for different observational scenarios; these are then used as inputs for the NEMESIS atmospheric retrieval code and the retrieved atmospheric properties (temperature structure, composition and cloud properties) compared with the known input values, following the method of Barstow et al. (2013a). To correctly retrieve the temperature structure and composition of the atmosphere to within 2 σ, we find that we require: a single transit or eclipse of a hot Jupiter orbiting a sun-like (G2) star at 35 pc to constrain the terminator and dayside atmospheres; 20 transits or eclipses of a warm Jupiter orbiting a similar star; 10 transits/eclipses of a hot Neptune orbiting an M dwarf at 6 pc; and 30 transits or eclipses of a GJ1214b-like planet. 1. Introduction The Exoplanet Characterisation Observatory (EChO; Tinetti et al. 2012) was a proposed European Space Agency mission for the Cosmic Visions M3 launch. Its goal was to survey the atmospheres of a range of extrasolar planets from the L2 Lagrange Point, over a period of 5 years.