ATLAS Deliverable 3.2
Total Page:16
File Type:pdf, Size:1020Kb
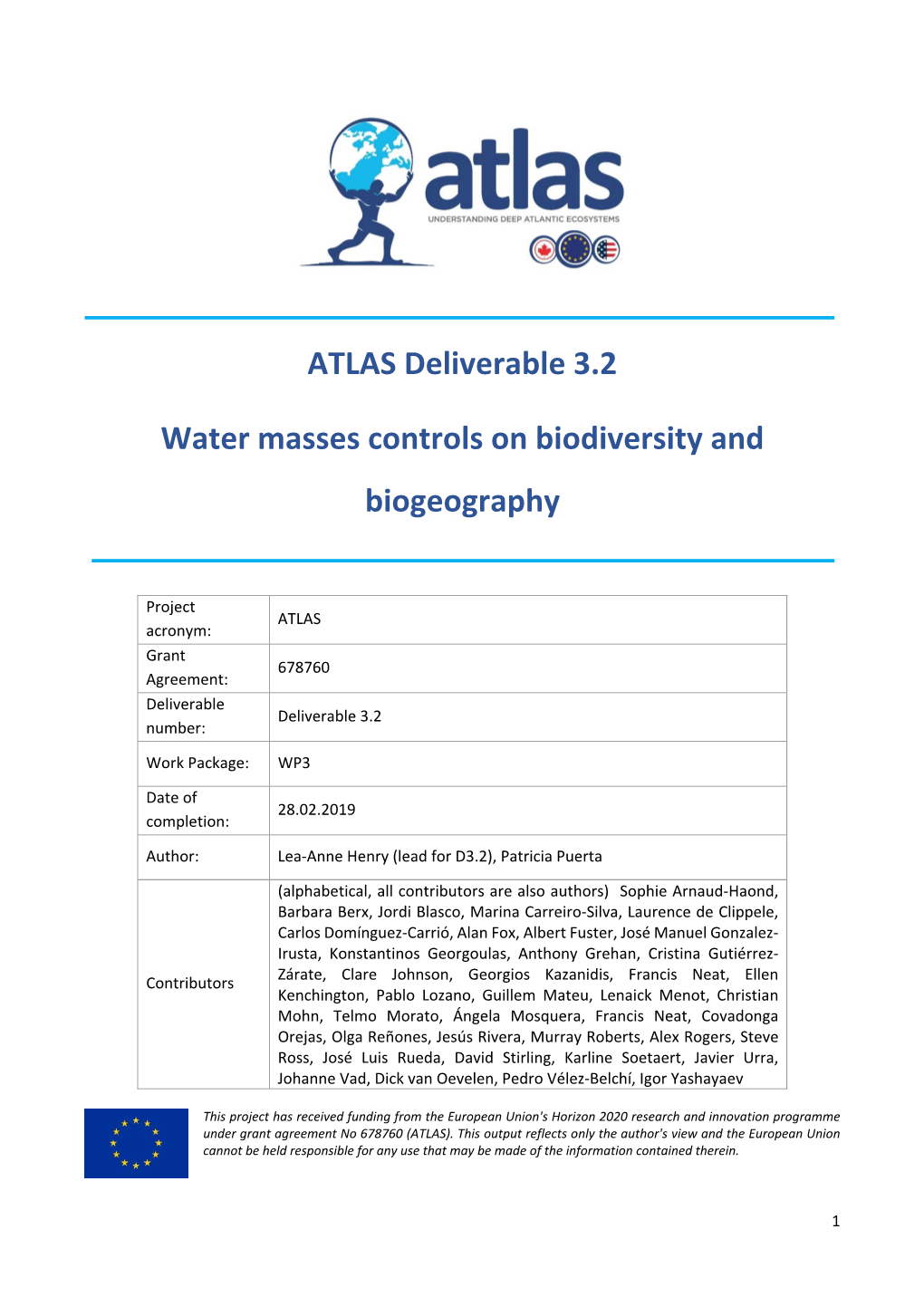
Load more
Recommended publications
-
Taxonomy and Diversity of the Sponge Fauna from Walters Shoal, a Shallow Seamount in the Western Indian Ocean Region
Taxonomy and diversity of the sponge fauna from Walters Shoal, a shallow seamount in the Western Indian Ocean region By Robyn Pauline Payne A thesis submitted in partial fulfilment of the requirements for the degree of Magister Scientiae in the Department of Biodiversity and Conservation Biology, University of the Western Cape. Supervisors: Dr Toufiek Samaai Prof. Mark J. Gibbons Dr Wayne K. Florence The financial assistance of the National Research Foundation (NRF) towards this research is hereby acknowledged. Opinions expressed and conclusions arrived at, are those of the author and are not necessarily to be attributed to the NRF. December 2015 Taxonomy and diversity of the sponge fauna from Walters Shoal, a shallow seamount in the Western Indian Ocean region Robyn Pauline Payne Keywords Indian Ocean Seamount Walters Shoal Sponges Taxonomy Systematics Diversity Biogeography ii Abstract Taxonomy and diversity of the sponge fauna from Walters Shoal, a shallow seamount in the Western Indian Ocean region R. P. Payne MSc Thesis, Department of Biodiversity and Conservation Biology, University of the Western Cape. Seamounts are poorly understood ubiquitous undersea features, with less than 4% sampled for scientific purposes globally. Consequently, the fauna associated with seamounts in the Indian Ocean remains largely unknown, with less than 300 species recorded. One such feature within this region is Walters Shoal, a shallow seamount located on the South Madagascar Ridge, which is situated approximately 400 nautical miles south of Madagascar and 600 nautical miles east of South Africa. Even though it penetrates the euphotic zone (summit is 15 m below the sea surface) and is protected by the Southern Indian Ocean Deep- Sea Fishers Association, there is a paucity of biodiversity and oceanographic data. -
Coastal and Marine Ecological Classification Standard (2012)
FGDC-STD-018-2012 Coastal and Marine Ecological Classification Standard Marine and Coastal Spatial Data Subcommittee Federal Geographic Data Committee June, 2012 Federal Geographic Data Committee FGDC-STD-018-2012 Coastal and Marine Ecological Classification Standard, June 2012 ______________________________________________________________________________________ CONTENTS PAGE 1. Introduction ..................................................................................................................... 1 1.1 Objectives ................................................................................................................ 1 1.2 Need ......................................................................................................................... 2 1.3 Scope ........................................................................................................................ 2 1.4 Application ............................................................................................................... 3 1.5 Relationship to Previous FGDC Standards .............................................................. 4 1.6 Development Procedures ......................................................................................... 5 1.7 Guiding Principles ................................................................................................... 7 1.7.1 Build a Scientifically Sound Ecological Classification .................................... 7 1.7.2 Meet the Needs of a Wide Range of Users ...................................................... -
A New Classification of the Chirostyloidea (Crustacea: Decapoda: Anomura)
Zootaxa 2687: 56–64 (2010) ISSN 1175-5326 (print edition) www.mapress.com/zootaxa/ Article ZOOTAXA Copyright © 2010 · Magnolia Press ISSN 1175-5334 (online edition) A new classification of the Chirostyloidea (Crustacea: Decapoda: Anomura) KAREEN E. SCHNABEL1 & SHANE T. AHYONG2 1National Institute of Water and Atmospheric Research, Private Bag 14901, Kilbirnie, Wellington, New Zealand. E-mail: [email protected] 2Australian Museum, 6 College Street, Sydney, NSW 2010 Australia. E-mail: [email protected] Abstract The high level classification of the Chirostyloidea Ortmann, 1892, is reviewed. Eumunididae Milne-Edwards & Bouvier, 1900, is resurrected for two genera formerly placed in the Chirostylidae Ortmann, 1892, Eumunida Smith, 1883, and Pseudomunida Haig, 1979, based on shared characteristics such as the dorsal carapace striation, presence of supraocular spines of the rostrum, dentition of the mandible, presence of an epipod and an annulated exopod flagellum of maxilliped 1. Three families are now included in the Chirostyloidea: Chirostylidae, Eumunididae and Kiwaidae. Diagnoses are provided for each family as well as a key to the families. The fossil record of the Chirostyloidea is discussed, with putative records of Eumunida in the fossil record referred to the galatheid genus Sadayoshia Baba, 1969. Key words: Galatheoidea, Chirostylidae, Eumunididae, Kiwaidae, adult somatic morphology, larval morphology, fossil record Introduction Recent focus on the phylogeny of Anomura has generated significant molecular phylogenetic information that has challenged the traditional understanding of the marine squat lobsters and porcelain crabs, the Galatheoidea, which comprised the Chirostylidae Ortmann, 1892, Galatheidae Samouelle, 1819, Porcellanidae Haworth, 1825, and Kiwaidae Macpherson, Jones & Segonzac, 2005 (e.g., Ahyong et al. -
Lab 8: Arthropoda OEB 51 Lab 8: Arthropoda
Lab 8: Arthropoda OEB 51 Lab 8: Arthropoda 6 April 2016 ATTENTION: Today we will be examining several preserved specimens from along the arthropod diversity and some exciting live specimens that you have not seen in the fieldtrip! Please keep all preserved specimens submerged. Examine using forceps and probes – be careful not to damage or remove the limbs of any specimen. And remember to return the specimen to its original spot in the lateral bench so your friends can find the animals properly labeled. • For each specimen, even when specific instructions are not given, make detailed observations and drawings and take notes on important characters in the evolution of arthropods, such as tagmata and adaptations to the specific environment where the organism lives. 1 Lab 8: Arthropoda OEB 51 Myriapoda With these living myriapods, take the opportunity to make a few notes on behavior – locomotory, hygienic (they frequently clean their antennae). CHOOSE ONE • Chilopoda (live) Do not handle these centipedes directly, as they are venomous (not deadly, but still). Watch these animals move, but don’t let them escape! Try to draw if you can. Compare to the large, preserved Scolopendra gigantea (MCZ specimen). Draw from the preserved for more morphological details. • Diplopoda (live) Contrary to centipedes, millipedes are docile (but they have deterrent substances, like cyanide compounds, which you might be able to smell after handling the specimens). Observe their movement. Make a sketch of the whole body and drawings of specific parts in more detail. • What is the most conspicuous difference between these two groups of myriapods? 2 Lab 8: Arthropoda OEB 51 Pycnogonida • Colossendeis colossea (Museum specimens, handle with care) • What characteristics of pycnogonids are not found in other arthropods? 3 Lab 8: Arthropoda OEB 51 Chelicerata • Xiphosura – Limulus (horseshoe crab). -
Biodiversity of the Kermadec Islands and Offshore Waters of the Kermadec Ridge: Report of a Coastal, Marine Mammal and Deep-Sea Survey (TAN1612)
Biodiversity of the Kermadec Islands and offshore waters of the Kermadec Ridge: report of a coastal, marine mammal and deep-sea survey (TAN1612) New Zealand Aquatic Environment and Biodiversity Report No. 179 Clark, M.R.; Trnski, T.; Constantine, R.; Aguirre, J.D.; Barker, J.; Betty, E.; Bowden, D.A.; Connell, A.; Duffy, C.; George, S.; Hannam, S.; Liggins, L..; Middleton, C.; Mills, S.; Pallentin, A.; Riekkola, L.; Sampey, A.; Sewell, M.; Spong, K.; Stewart, A.; Stewart, R.; Struthers, C.; van Oosterom, L. ISSN 1179-6480 (online) ISSN 1176-9440 (print) ISBN 978-1-77665-481-9 (online) ISBN 978-1-77665-482-6 (print) January 2017 Requests for further copies should be directed to: Publications Logistics Officer Ministry for Primary Industries PO Box 2526 WELLINGTON 6140 Email: [email protected] Telephone: 0800 00 83 33 Facsimile: 04-894 0300 This publication is also available on the Ministry for Primary Industries websites at: http://www.mpi.govt.nz/news-resources/publications.aspx http://fs.fish.govt.nz go to Document library/Research reports © Crown Copyright - Ministry for Primary Industries TABLE OF CONTENTS EXECUTIVE SUMMARY 1 1. INTRODUCTION 3 1.1 Objectives: 3 1.2 Objective 1: Benthic offshore biodiversity 3 1.3 Objective 2: Marine mammal research 4 1.4 Objective 3: Coastal biodiversity and connectivity 5 2. METHODS 5 2.1 Survey area 5 2.2 Survey design 6 Offshore Biodiversity 6 Marine mammal sampling 8 Coastal survey 8 Station recording 8 2.3 Sampling operations 8 Multibeam mapping 8 Photographic transect survey 9 Fish and Invertebrate sampling 9 Plankton sampling 11 Catch processing 11 Environmental sampling 12 Marine mammal sampling 12 Dive sampling operations 12 Outreach 13 3. -
Deep-Sea Origin and In-Situ Diversification of Chrysogorgiid Octocorals
Deep-Sea Origin and In-Situ Diversification of Chrysogorgiid Octocorals Eric Pante1*¤, Scott C. France1, Arnaud Couloux2, Corinne Cruaud2, Catherine S. McFadden3, Sarah Samadi4, Les Watling5,6 1 Department of Biology, University of Louisiana at Lafayette, Lafayette, Louisiana, United States of America, 2 GENOSCOPE, Centre National de Se´quenc¸age, Evry, France, 3 Department of Biology, Harvey Mudd College, Claremont, California, United States of America, 4 De´partement Syste´matique et Evolution, UMR 7138 UPMC-IRD-MNHN- CNRS (UR IRD 148), Muse´um national d’Histoire naturelle, Paris, France, 5 Department of Biology, University of Hawaii at Manoa, Honolulu, Hawaii, United States of America, 6 Darling Marine Center, University of Maine, Walpole, Maine, United States of America Abstract The diversity, ubiquity and prevalence in deep waters of the octocoral family Chrysogorgiidae Verrill, 1883 make it noteworthy as a model system to study radiation and diversification in the deep sea. Here we provide the first comprehensive phylogenetic analysis of the Chrysogorgiidae, and compare phylogeny and depth distribution. Phylogenetic relationships among 10 of 14 currently-described Chrysogorgiidae genera were inferred based on mitochondrial (mtMutS, cox1) and nuclear (18S) markers. Bathymetric distribution was estimated from multiple sources, including museum records, a literature review, and our own sampling records (985 stations, 2345 specimens). Genetic analyses suggest that the Chrysogorgiidae as currently described is a polyphyletic family. Shallow-water genera, and two of eight deep-water genera, appear more closely related to other octocoral families than to the remainder of the monophyletic, deep-water chrysogorgiid genera. Monophyletic chrysogorgiids are composed of strictly (Iridogorgia Verrill, 1883, Metallogorgia Versluys, 1902, Radicipes Stearns, 1883, Pseudochrysogorgia Pante & France, 2010) and predominantly (Chrysogorgia Duchassaing & Michelotti, 1864) deep-sea genera that diversified in situ. -
DEEP SEA LEBANON RESULTS of the 2016 EXPEDITION EXPLORING SUBMARINE CANYONS Towards Deep-Sea Conservation in Lebanon Project
DEEP SEA LEBANON RESULTS OF THE 2016 EXPEDITION EXPLORING SUBMARINE CANYONS Towards Deep-Sea Conservation in Lebanon Project March 2018 DEEP SEA LEBANON RESULTS OF THE 2016 EXPEDITION EXPLORING SUBMARINE CANYONS Towards Deep-Sea Conservation in Lebanon Project Citation: Aguilar, R., García, S., Perry, A.L., Alvarez, H., Blanco, J., Bitar, G. 2018. 2016 Deep-sea Lebanon Expedition: Exploring Submarine Canyons. Oceana, Madrid. 94 p. DOI: 10.31230/osf.io/34cb9 Based on an official request from Lebanon’s Ministry of Environment back in 2013, Oceana has planned and carried out an expedition to survey Lebanese deep-sea canyons and escarpments. Cover: Cerianthus membranaceus © OCEANA All photos are © OCEANA Index 06 Introduction 11 Methods 16 Results 44 Areas 12 Rov surveys 16 Habitat types 44 Tarablus/Batroun 14 Infaunal surveys 16 Coralligenous habitat 44 Jounieh 14 Oceanographic and rhodolith/maërl 45 St. George beds measurements 46 Beirut 19 Sandy bottoms 15 Data analyses 46 Sayniq 15 Collaborations 20 Sandy-muddy bottoms 20 Rocky bottoms 22 Canyon heads 22 Bathyal muds 24 Species 27 Fishes 29 Crustaceans 30 Echinoderms 31 Cnidarians 36 Sponges 38 Molluscs 40 Bryozoans 40 Brachiopods 42 Tunicates 42 Annelids 42 Foraminifera 42 Algae | Deep sea Lebanon OCEANA 47 Human 50 Discussion and 68 Annex 1 85 Annex 2 impacts conclusions 68 Table A1. List of 85 Methodology for 47 Marine litter 51 Main expedition species identified assesing relative 49 Fisheries findings 84 Table A2. List conservation interest of 49 Other observations 52 Key community of threatened types and their species identified survey areas ecological importanc 84 Figure A1. -
Updated Checklist of Marine Fishes (Chordata: Craniata) from Portugal and the Proposed Extension of the Portuguese Continental Shelf
European Journal of Taxonomy 73: 1-73 ISSN 2118-9773 http://dx.doi.org/10.5852/ejt.2014.73 www.europeanjournaloftaxonomy.eu 2014 · Carneiro M. et al. This work is licensed under a Creative Commons Attribution 3.0 License. Monograph urn:lsid:zoobank.org:pub:9A5F217D-8E7B-448A-9CAB-2CCC9CC6F857 Updated checklist of marine fishes (Chordata: Craniata) from Portugal and the proposed extension of the Portuguese continental shelf Miguel CARNEIRO1,5, Rogélia MARTINS2,6, Monica LANDI*,3,7 & Filipe O. COSTA4,8 1,2 DIV-RP (Modelling and Management Fishery Resources Division), Instituto Português do Mar e da Atmosfera, Av. Brasilia 1449-006 Lisboa, Portugal. E-mail: [email protected], [email protected] 3,4 CBMA (Centre of Molecular and Environmental Biology), Department of Biology, University of Minho, Campus de Gualtar, 4710-057 Braga, Portugal. E-mail: [email protected], [email protected] * corresponding author: [email protected] 5 urn:lsid:zoobank.org:author:90A98A50-327E-4648-9DCE-75709C7A2472 6 urn:lsid:zoobank.org:author:1EB6DE00-9E91-407C-B7C4-34F31F29FD88 7 urn:lsid:zoobank.org:author:6D3AC760-77F2-4CFA-B5C7-665CB07F4CEB 8 urn:lsid:zoobank.org:author:48E53CF3-71C8-403C-BECD-10B20B3C15B4 Abstract. The study of the Portuguese marine ichthyofauna has a long historical tradition, rooted back in the 18th Century. Here we present an annotated checklist of the marine fishes from Portuguese waters, including the area encompassed by the proposed extension of the Portuguese continental shelf and the Economic Exclusive Zone (EEZ). The list is based on historical literature records and taxon occurrence data obtained from natural history collections, together with new revisions and occurrences. -
The Lower Bathyal and Abyssal Seafloor Fauna of Eastern Australia T
O’Hara et al. Marine Biodiversity Records (2020) 13:11 https://doi.org/10.1186/s41200-020-00194-1 RESEARCH Open Access The lower bathyal and abyssal seafloor fauna of eastern Australia T. D. O’Hara1* , A. Williams2, S. T. Ahyong3, P. Alderslade2, T. Alvestad4, D. Bray1, I. Burghardt3, N. Budaeva4, F. Criscione3, A. L. Crowther5, M. Ekins6, M. Eléaume7, C. A. Farrelly1, J. K. Finn1, M. N. Georgieva8, A. Graham9, M. Gomon1, K. Gowlett-Holmes2, L. M. Gunton3, A. Hallan3, A. M. Hosie10, P. Hutchings3,11, H. Kise12, F. Köhler3, J. A. Konsgrud4, E. Kupriyanova3,11,C.C.Lu1, M. Mackenzie1, C. Mah13, H. MacIntosh1, K. L. Merrin1, A. Miskelly3, M. L. Mitchell1, K. Moore14, A. Murray3,P.M.O’Loughlin1, H. Paxton3,11, J. J. Pogonoski9, D. Staples1, J. E. Watson1, R. S. Wilson1, J. Zhang3,15 and N. J. Bax2,16 Abstract Background: Our knowledge of the benthic fauna at lower bathyal to abyssal (LBA, > 2000 m) depths off Eastern Australia was very limited with only a few samples having been collected from these habitats over the last 150 years. In May–June 2017, the IN2017_V03 expedition of the RV Investigator sampled LBA benthic communities along the lower slope and abyss of Australia’s eastern margin from off mid-Tasmania (42°S) to the Coral Sea (23°S), with particular emphasis on describing and analysing patterns of biodiversity that occur within a newly declared network of offshore marine parks. Methods: The study design was to deploy a 4 m (metal) beam trawl and Brenke sled to collect samples on soft sediment substrata at the target seafloor depths of 2500 and 4000 m at every 1.5 degrees of latitude along the western boundary of the Tasman Sea from 42° to 23°S, traversing seven Australian Marine Parks. -
Proceedings of the United States National Museum
descriptions of a new genus and forty-six new spp:cies of crusta(jeans of the family GALA- theid.e, with a list of the known marine SPECIES. By James E. Benedict, Assistant Curator of Marine Invertebrates. The collection of Galatheids in the United States National Museum, upon which this paper is based, began with the first dredgings of the U. S. Fish Commission steamer Albatross in 1883, and has grown as that busy ship has had opportunit}' to dredge. During the first period of its work many of the species taken were identical with those found by the U. S. Coast Survey steamer JBlake, afterwards described by A. Milne-Edwards, and in addition several new species were collected. During the voyage of the Albatross to the Pacific Ocean through the Straits of Magellan interesting addi- tions were made to the collection. Since then the greater part of the time spent by the Albatross at sea has been in Alaskan waters, where Galatheids do not seem to abound. However, occasional cruises else- where have greatly enriched the collection, notably three—one in the Gulf of California, one to the Galapagos Islands, and one to the coast of Japan and southward. The U. S. National Museum has received a number of specimens from the Museum of Natural History, Paris, and also from the Indian Museum, Calcutta. The literature of the deep-sea Galatheidie from the nature of the case is not greatly scattered. The first considerate number of species were described by A. Milne-Edwards from dredgings made by the Blake in the West Indian region. -
Evolutionary Relationships Within the "Bathymodiolus" Childressi Group
Cah. Biol. Mar. (2006) 47 : 403-407 Evolutionary relationships within the "Bathymodiolus" childressi group W. Jo JONES* and Robert C. VRIJENHOEK Monterey Bay Aquarium Research Institute, Moss Landing CA 95064, USA, *Corresponding Author: Phone: 831-775-1789, Fax: 831-775-1620, E-mail: [email protected] Abstract: Recent discoveries of deep-sea mussel species from reducing environments have revealed a much broader phylogenetic diversity than previously imagined. In this study, we utilize a commercially available DNA extraction kit to obtain high-quality DNA from two mussel shells collected eight years ago at the Edison Seamount near Papua New Guinea. We include these two species into a comprehensive phylogeny of all available deep-sea mussels. Our analysis of nuclear and mitochondrial DNA sequences supports previous conclusions that deep-sea mussels presently subsumed within the genus Bathymodiolus comprise a paraphyletic assemblage. This assemblage is composed of a monophyletic group that might properly be called Bathymodiolus and a distinctly parallel grouping that we refer to as the “Bathymodiolus” childressi clade. The “childressi” clade itself is diverse containing species from the western Pacific and Atlantic basins. Keywords: Bathymodiolus l Phylogeny l Childressi clade l Deep-sea l Mussel Introduction example, Gustafson et al. (1998) noted that “Bathymodiolus” childressi Gustafson et al. (their quotes), Many new species of mussels (Bivalvia: Mytilidae: a newly discovered species from the Gulf of Mexico, Bathymodiolinae) have been discovered during the past differed from other known Bathymodiolus for a number of two decades of deep ocean exploration. A number of genus morphological characters: multiple separation of posterior names are currently applied to members of this subfamily byssal retractors, single posterior byssal retractor scar, and (e.g., Adipicola, Bathymodiolus, Benthomodiolus, rectum that enters ventricle posterior to level of auricular Gigantidas, Idas, Myrina and Tamu), but diagnostic ostia. -
Deep-Water Squat Lobsters (Crustacea: Decapoda: Anomura) from India Collected by the FORV Sagar Sampada
Bull. Natl. Mus. Nat. Sci., Ser. A, 46(4), pp. 155–182, November 20, 2020 Deep-water Squat Lobsters (Crustacea: Decapoda: Anomura) from India Collected by the FORV Sagar Sampada Vinay P. Padate1, 2, Shivam Tiwari1, 3, Sherine Sonia Cubelio1,4 and Masatsune Takeda5 1Centre for Marine Living Resources and Ecology, Ministry of Earth Sciences, Government of India. Atal Bhavan, LNG Terminus Road, Puthuvype, Kochi 682508, India 2Corresponding author: [email protected]; https://orcid.org/0000-0002-2244-8338 [email protected]; https://orcid.org/0000-0001-6194-8960 [email protected]; http://orcid.org/0000-0002-2960-7055 5Department of Zoology, National Museum of Nature and Science, Tokyo. 4–1–1 Amakubo, Tsukuba, Ibaraki 305–0005, Japan. [email protected]; https://orcid/org/0000-0002-0028-1397 (Received 13 August 2020; accepted 23 September 2020) Abstract Deep-water squat lobsters collected during five cruises of the Fishery Oceanographic Research Vessel Sagar Sampada off the Andaman and Nicobar Archipelagos (299–812 m deep) and three cruises in the southeastern Arabian Sea (610–957 m deep) are identified. They are referred to each one species of the families Chirostylidae and Sternostylidae in the Superfamily Chirostyloidea, and five species of the family Munidopsidae and three species of the family Muni- didae in the Superfamily Galatheoidea. Of altogether 10 species of 5 genera dealt herein, the Uro- ptychus species of the Chirostylidae is described as new to science, and Agononida aff. indocerta Poore and Andreakis, 2012, of the Munididae, previously reported from Western Australia and Papua New Guinea, is newly recorded from Indian waters.