Morphogenetic Movements Underlying Eye Field Formation Require Interactions Between the FGF and Ephrinb1 Signaling Pathways
Total Page:16
File Type:pdf, Size:1020Kb
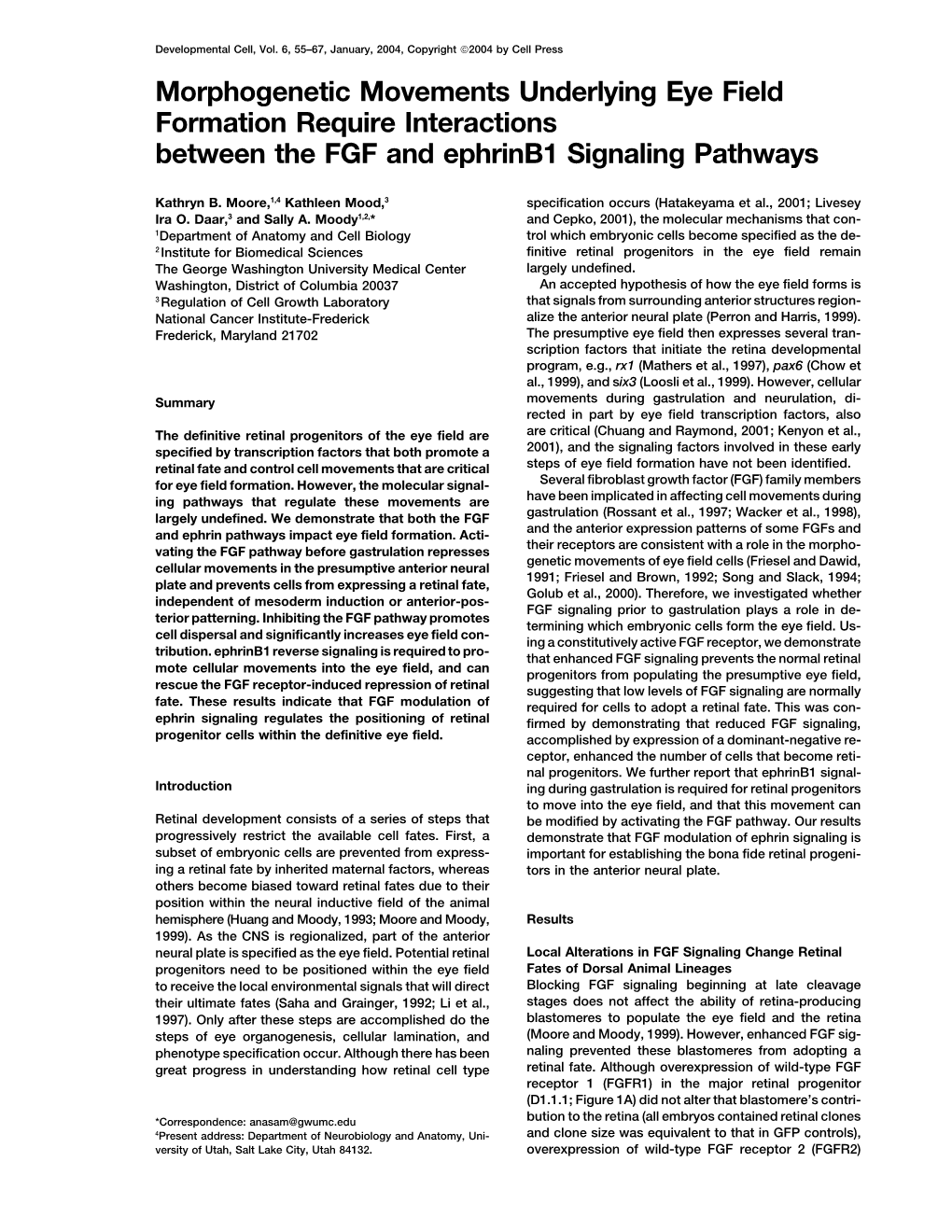
Load more
Recommended publications
-
Rabbit Anti-Ephrin-A1 Rabbit Anti-Ephrin-A1
Qty: 100 µg/400 µl Rabbit anti-ephrin-A1 Catalog No. 34-3300 Lot No. See product label Rabbit anti-ephrin-A1 FORM This polyclonal antibody is supplied as a 400 µl aliquot at a concentration of 0.25 mg/ml in phosphate buffered saline (pH 7.4) containing 0.1% sodium azide. The antibody is epitope-affinity-purified from rabbit antiserum. PAD: ZMD.39 IMMUNOGEN Synthetic peptide derived from the C-terminal end of mouse ephrin-A1 protein. SPECIFICITY This antibody is specific for the ephrin-A1 protein. REACTIVITY Reactivity is confirmed with a chimeric protein consisting of the extracellular domain of mouse ephrin-A1 and the Fc region of human IgG1. Cross-reactivity with human ephrin-A1 is confirmed with IHC experiments on human tissue sections and this reactivity was expected because of the 85% shared amino acid identity in the extracellular domain. Sample Western Immunoprecipitation Immunohistochemistry Blotting (FFPE) Mouse +++ +++ NT Human NT NT ++ (Excellent +++, Good++, Poor +, No reactivity 0, Not tested NT) USAGE Working concentrations for specific applications should be determined by the investigator. Appropriate concentrations will be affected by several factors, including secondary antibody affinity, antigen concentration, sensitivity of detection method, temperature and length of incubations, etc. The suitability of this antibody for applications other than those listed below has not been determined. The following concentration ranges are recommended starting points for this product. Western Blotting: 1-5 µg/mL Immunoprecipitation: 5-10 µg/ IP reaction Immunohistochemistry: 4-10 µg/mL Please note that immunohistochemical assays were optimized on formalin-fixed, paraffin-embedded tissue sections and Heat Induced Epitope Retrieval (HIER) with EDTA, pH 8.0 was required for optimal staining. -
EPH/Ephrin Profile and EPHB2 Expression Predicts Patient Survival in Breast Cancer
www.impactjournals.com/oncotarget/ Oncotarget, Vol. 7, No. 16 EPH/ephrin profile and EPHB2 expression predicts patient survival in breast cancer Anna-Maria Husa1,2, Željana Magić1, Malin Larsson3, Tommy Fornander4, Gizeh Pérez-Tenorio1 1Department of Clinical and Experimental Medicine, Division of Oncology, Linköping University, Linköping, Sweden 2Current address: CCRI, Children’s Cancer Research Institute, St. Anna Kinderkrebsforschung e.V., Vienna, Austria 3Bioinformatics Infrastructure for Life Sciences (BILS) and Department of Physics, Chemistry and Biology, Linköping University, Linköping, Sweden 4Department of Oncology, Karolinska University Hospital and Karolinska Institute, Stockholm, Sweden Correspondence to: Gizeh Pérez-Tenorio, e-mail: [email protected] Keywords: EPHB2, EPH family, TaqMan array, gene expression, protein expression Received: July 03, 2015 Accepted: January 23, 2016 Published: February 8, 2016 ABSTRACT The EPH and ephrins function as both receptor and ligands and the output on their complex signaling is currently investigated in cancer. Previous work shows that some EPH family members have clinical value in breast cancer, suggesting that this family could be a source of novel clinical targets. Here we quantified the mRNA expression levels of EPH receptors and their ligands, ephrins, in 65 node positive breast cancer samples by RT-PCR with TaqMan® Micro Fluidics Cards Microarray. Upon hierarchical clustering of the mRNA expression levels, we identified a subgroup of patients with high expression, and poor clinical outcome. EPHA2, EPHA4, EFNB1, EFNB2, EPHB2 and EPHB6 were significantly correlated with the cluster groups and particularly EPHB2 was an independent prognostic factor in multivariate analysis and in four public databases. The EPHB2 protein expression was also analyzed by immunohistochemistry in paraffin embedded material (cohort 2). -
Molecular Regulation of Visual System Development: More Than Meets the Eye
Downloaded from genesdev.cshlp.org on September 30, 2021 - Published by Cold Spring Harbor Laboratory Press REVIEW Molecular regulation of visual system development: more than meets the eye Takayuki Harada,1,2 Chikako Harada,1,2 and Luis F. Parada1,3 1Department of Developmental Biology and Kent Waldrep Foundation Center for Basic Neuroscience Research on Nerve Growth and Regeneration, University of Texas Southwestern Medical Center, Dallas, Texas 75235, USA; 2Department of Molecular Neurobiology, Tokyo Metropolitan Institute for Neuroscience, Fuchu, Tokyo 183-8526, Japan Vertebrate eye development has been an excellent model toderm, intercalating mesoderm, surface ectoderm, and system to investigate basic concepts of developmental neural crest (Fig. 1). The neuroectoderm differentiates biology ranging from mechanisms of tissue induction to into the retina, iris, and optic nerve; the surface ecto- the complex patterning and bidimensional orientation of derm gives rise to lens and corneal epithelium; the me- the highly specialized retina. Recent advances have shed soderm differentiates into the extraocular muscles and light on the interplay between numerous transcriptional the fibrous and vascular coats of the eye; and neural crest networks and growth factors that are involved in the cells become the corneal stroma sclera and corneal en- specific stages of retinogenesis, optic nerve formation, dothelium. The vertebrate eye originates from bilateral and topographic mapping. In this review, we summarize telencephalic optic grooves. In humans, optic vesicles this recent progress on the molecular mechanisms un- emerge at the end of the fourth week of development and derlying the development of the eye, visual system, and soon thereafter contact the surface ectoderm to induce embryonic tumors that arise in the optic system. -
Migration Dichotomy of Glioblastoma by Interacting with Focal Adhesion Kinase
Oncogene (2012) 31, 5132 --5143 & 2012 Macmillan Publishers Limited All rights reserved 0950-9232/12 www.nature.com/onc ORIGINAL ARTICLE EphB2 receptor controls proliferation/migration dichotomy of glioblastoma by interacting with focal adhesion kinase SD Wang1, P Rath1,2, B Lal1,2, J-P Richard1,2,YLi1,2, CR Goodwin3, J Laterra1,2,4,5 and S Xia1,2 Glioblastoma multiforme (GBM) is the most frequent and aggressive primary brain tumors in adults. Uncontrolled proliferation and abnormal cell migration are two prominent spatially and temporally disassociated characteristics of GBMs. In this study, we investigated the role of the receptor tyrosine kinase EphB2 in controlling the proliferation/migration dichotomy of GBM. We studied EphB2 gain of function and loss of function in glioblastoma-derived stem-like neurospheres, whose in vivo growth pattern closely replicates human GBM. EphB2 expression stimulated GBM neurosphere cell migration and invasion, and inhibited neurosphere cell proliferation in vitro. In parallel, EphB2 silencing increased tumor cell proliferation and decreased tumor cell migration. EphB2 was found to increase tumor cell invasion in vivo using an internally controlled dual-fluorescent xenograft model. Xenografts derived from EphB2-overexpressing GBM neurospheres also showed decreased cellular proliferation. The non-receptor tyrosine kinase focal adhesion kinase (FAK) was found to be co-associated with and highly activated by EphB2 expression, and FAK activation facilitated focal adhesion formation, cytoskeleton structure change and cell migration in EphB2-expressing GBM neurosphere cells. Taken together, our findings indicate that EphB2 has pro-invasive and anti-proliferative actions in GBM stem-like neurospheres mediated, in part, by interactions between EphB2 receptors and FAK. -
Regulation of Ephb2 Activation and Cell Repulsion by Feedback Control Of
JCB: ARTICLE Regulation of EphB2 activation and cell repulsion by feedback control of the MAPK pathway Alexei Poliakov , Maria L. Cotrina , Andrea Pasini , and David G. Wilkinson Division of Developmental Neurobiology, Medical Research Council National Institute for Medical Research, London NW7 1AA, England, UK n this study, we investigated whether the ability of Eph phosphorylation of EphB2 upon stimulation with ephrinB1, receptor signaling to mediate cell repulsion is antago- and we show that this involves a requirement for the I nized by fi broblast growth factor receptor (FGFR) acti- mitogen-activated protein kinase (MAPK) pathway. In the vation that can promote cell invasion. We fi nd that absence of activated FGFR1, EphB2 activates the MAPK activation of FGFR1 in EphB2-expressing cells prevents pathway, which in turn promotes EphB2 activation in a segregation, repulsion, and collapse responses to ephrinB1 positive feedback loop. However, after FGFR1 activation, ligand. FGFR1 activation leads to increased phosphoryla- the induction of Sprouty genes inhibits the MAPK pathway tion of unstimulated EphB2, which we show is caused downstream of EphB2 and decreases cell repulsion and by down-regulation of the leukocyte common antigen – segregation. These fi ndings reveal a novel feedback loop related tyrosine phosphatase receptor that dephosphory- that promotes EphB2 activation and cell repulsion that is lates EphB2. In addition, FGFR1 signaling inhibits further blocked by transcriptional targets of FGFR1. Introduction The control of cell movement is essential for the establishment gration can occur by convergence of downstream pathways, for and maintenance of tissue organization during embryogenesis. example on central components of cytoskeletal regulation, and/or For example, mixing of cell populations that have distinct by interactions between distinct receptors that modulate each regional or tissue identity is prevented by inhibition of cell mi- others ’ activity ( Huber et al., 2003 ). -
Neurosurgical Focus Neurosurg Focus 38 (5):E2, 2015
NEUROSURGICAL FOCUS Neurosurg Focus 38 (5):E2, 2015 Insights into the development of molecular therapies for craniosynostosis Jennifer Kosty, MD,1 and Timothy W. Vogel, MD2 1Department of Neurosurgery, University of Cincinnati; and 2Department of Neurosurgery, Cincinnati Children’s Hospital and Medical Center, Cincinnati, Ohio For the past 2 decades, clinical and basic science researchers have gained significant insights into the molecular and genetic pathways associated with common forms of craniosynostosis. This has led to invaluable information for families and physicians in their attempts to understand the heterogeneity of craniosynostosis. Genetic mutations have been iden- tified in the fibroblast growth factor receptors (FGFRs) as well as in other targets, including TWIST1, BMP, and RUNX2. Greater understanding of these and other pathways has led to the development of innovative approaches for applying medical therapies to the treatment of craniosynostosis, in particular by maintaining suture patency. In this article, the authors discuss the molecular pathophysiological mechanisms underlying various forms of craniosynostosis. They also highlight recent developments in the field of molecular craniosynostosis research with the hope of identifying targets for medical therapies that might augment the results of surgical intervention. http://thejns.org/doi/abs/10.3171/2015.2.FOCUS155 KEY WORDS craniosynostosis; fibroblast growth factor receptor; TWIST; genetics; molecular mechanisms; suture biology; therapy N 1993, Jabs et al.11 were -
Erbb2, Ephrinb1, Src Kinase and PTPN13 Signaling Complex Regulates MAP Kinase Signaling in Human Cancers
View metadata, citation and similar papers at core.ac.uk brought to you by CORE provided by Harvard University - DASH ErbB2, EphrinB1, Src Kinase and PTPN13 Signaling Complex Regulates MAP Kinase Signaling in Human Cancers The Harvard community has made this article openly available. Please share how this access benefits you. Your story matters. Citation Vermeer, Paola D., Megan Bell, Kimberly Lee, Daniel W. Vermeer, Byrant G. Wieking, Erhan Bilal, Gyan Bhanot, et al. 2012. ErbB2, EphrinB1, Src kinase and PTPN13 signaling complex regulates map kinase signaling in human cancers. PLoS ONE 7(1): e30447. Published Version doi:10.1371/journal.pone.0030447 Accessed February 19, 2015 9:51:50 AM EST Citable Link http://nrs.harvard.edu/urn-3:HUL.InstRepos:10288634 Terms of Use This article was downloaded from Harvard University's DASH repository, and is made available under the terms and conditions applicable to Other Posted Material, as set forth at http://nrs.harvard.edu/urn-3:HUL.InstRepos:dash.current.terms-of- use#LAA (Article begins on next page) ErbB2, EphrinB1, Src Kinase and PTPN13 Signaling Complex Regulates MAP Kinase Signaling in Human Cancers Paola D. Vermeer1, Megan Bell1, Kimberly Lee1, Daniel W. Vermeer1, Byrant G. Wieking1, Erhan Bilal2,Gyan Bhanot3, Ronny I. Drapkin4, Shridar Ganesan5, Aloysius J. Klingelhutz6,WiljanJ.Hendriks7,JohnH.Lee1,8* 1 Cancer Biology Research Center, Sanford Research/University of South Dakota, Sioux Falls, South Dakota, United States of America, 2 Thomas J. Watson Research Center, IBM Research, Yorktown -
Ephrin-B1 Signals Integrin-Mediated Responses 3075
Research Article 3073 Ephrin-B1 transduces signals to activate integrin- mediated migration, attachment and angiogenesis Uyen Huynh-Do1,2, Cécile Vindis2, Hua Liu1,3, Douglas Pat Cerretti3, Jeffrey T. McGrew3, Miriam Enriquez1, Jin Chen1,4,5,* and Thomas O. Daniel1,3 1Vanderbilt-Ingram Cancer Center, Departments of Medicine, Vanderbilt University Medical Center, Nashville, TN 37232, USA 2Division of Nephrology and Hypertension, Department of Medicine and Clinical Research, University of Bern, CH-3010 Bern, Switzerland 3Immunex Corporation, Seattle, WA 98101, USA 4Cancer Biology, Vanderbilt University Medical Center, Nashville, TN 37232 USA 5Cell Biology, Vanderbilt University Medical Center, Nashville, TN 37232, USA *Author for correspondence (e-mail: [email protected]) Accepted 16 May 2002 Journal of Cell Science 115, 3073-3081 (2002) © The Company of Biologists Ltd Summary Ephrin-B/EphB family proteins are implicated in p38 MAPkinases. By contrast, mutant ephrin-B1s bearing bidirectional signaling and were initially defined through either a cytoplasmic deletion (ephrin-B1∆Cy) or a deletion the function of their ectodomain sequences in activating of four C-terminal amino acids (ephrin-B1∆PDZbd) fail EphB receptor tyrosine kinases. Ephrin-B1-3 are to activate p46 JNK. Transient expression of intact transmembrane proteins sharing highly conserved C- ephin-B1 conferred EphB1/Fc migration responses on terminal cytoplasmic sequences. Here we use a soluble CHO cells, whereas the ephrin-B1∆Cy and ephrin- EphB1 ectodomain fusion protein (EphB1/Fc) to B1∆PDZbd mutants were inactive. Thus ephrin-B1 demonstrate that ephrin-B1 transduces signals that transduces ‘outside-in’ signals through C-terminal protein regulate cell attachment and migration. -
The Role of Eph Proteins in Haematopoiesis and Leukaemia
The role of Eph proteins in haematopoiesis and leukaemia Sara Charmsaz A thesis submitted for the degree of Doctor of Philosophy at The University of Queensland in 2014 The University of Queensland School of Medicine ABSTRACT Eph and their membrane bound ephrin ligands represent the largest family of receptor tyrosine kinases (RTKs). Ephs interact with cell-surface ephrin ligands to direct cell migration and orchestrate developmental patterning during embryogenesis by modulating cell shape and adhesion. Members of the Eph/ephrin family of RTKs are expressed in adult life and have ongoing roles both in normal tissue homeostasis and in responses to pathological situations. Eph/ephrin proteins are also expressed on many tumours including leukaemia, prostate cancer, colorectal cancer and brain cancer. High expression in a number of cancers has been linked to progression through facilitation of invasiveness and metastatic spread. Some members of the Eph/ephrin family of proteins are expressed on normal and cancerous haematopoietic cells. Real time PCR analysis showed expression of all EphA proteins on the haematopoietic stem cell (HSC) population, except for EphA6 and EphA8. I have used EphA1, EphA2 and EphA7 knockout mice to investigate the effect of these individual Eph proteins in normal haematopoiesis, and in particular haematopoietic stem cells in vivo. These studies showed that mice lacking EphA1, EphA2 or EphA7 have normal haematopoiesis and no significant defects were observed in HSCs. This suggests that if Eph receptors have a role in haematopoietic differentiation the presence of other Eph receptors may compensate for the absence of individual Eph proteins. EphA2 expression has been investigated in many different solid tumours and expression of this member of the Eph/ephrin family has been detected in mixed lineage leukaemia (MLL). -
Harnessing the Power of Eph/Ephrin Biosemiotics for Theranostic Applications
pharmaceuticals Review Harnessing the Power of Eph/ephrin Biosemiotics for Theranostic Applications Robert M. Hughes 1 and Jitka A.I. Virag 2,* 1 Department of Chemistry, East Carolina University, Science and Technology Building, SZ564, East 5th St, Greenville, NC 27858, USA; [email protected] 2 Department of Physiology, Brody School of Medicine, East Carolina University, Warren Life Sciences Building, LSB239, 600 Moye Blvd, Greenville, NC 27834, USA * Correspondence: [email protected] Received: 11 April 2020; Accepted: 27 May 2020; Published: 1 June 2020 Abstract: Comprehensive basic biological knowledge of the Eph/ephrin system in the physiologic setting is needed to facilitate an understanding of its role and the effects of pathological processes on its activity, thereby paving the way for development of prospective therapeutic targets. To this end, this review briefly addresses what is currently known and being investigated in order to highlight the gaps and possible avenues for further investigation to capitalize on their diverse potential. Keywords: Eph/ephrin; receptor tyrosine kinase; therapeutic target; smart drug; nanoparticles; liposomes; exosomes 1. Introduction Detection, transduction and appropriate response behavior of neighboring cells are essential components of optimal communication and the coordinated and integrated function necessary for survival. In a complex system, this is determined by the summative and timely intracellular processing of a myriad of intersecting signals from various distances and directions. The Eph receptors and ephrin ligands are the largest tyrosine kinase (RTK) superfamily, comprised of 22 known members, subdivided into A- and B-subclasses, and are heterogeneously expressed in almost every tissue [1]. These membrane-anchored proteins exhibit unique cell-to-cell communication via bidirectional signaling, which modulates cytoskeletal dynamics and thus cell–cell recognition and motility, making them integral mediators in developmental processes and the maintenance of homeostasis [2,3]. -
Eph Receptors and Ephrin Ligands: Embryogenesis to Tumorigenesis
Oncogene (2000) 19, 5614 ± 5619 ã 2000 Macmillan Publishers Ltd All rights reserved 0950 ± 9232/00 $15.00 www.nature.com/onc Eph receptors and ephrin ligands: embryogenesis to tumorigenesis Vincent C Dodelet1 and Elena B Pasquale*,1 1The Burnham Institute, 10901 North Torrey Pines Road, La Jolla, California, CA 92037, USA Protein tyrosine kinase genes are the largest family of The Eph receptors become phosphorylated at speci®c oncogenes. This is not surprising since tyrosine kinases tyrosine residues in the cytoplasmic domain following are important components of signal transduction path- ligand binding (Figure 1). Phosphorylated motifs serve ways that control cell shape, proliferation, dierentia- as sites of interaction with certain cytoplasmic signaling tion, and migration. At 14 distinct members, the Eph proteins such as non-receptor tyrosine kinases of the kinases constitute the largest family of receptor tyrosine Src and Abl families and the non-receptor phosphotyr- kinases. Although they have been most intensively osine phosphatase LMW ± PTP (low molecular weight studied for their roles in embryonic development, phosphotyrosine phosphatase); the enzymes phospholi- increasing evidence also implicates Eph family proteins pase C g, phosphatidylinositol 3-kinase, and Ras in cancer. This review will address the recent progress in GTPase activating protein; the adaptors SLAP, Grb2, understanding the function of Eph receptors in normal Grb10, and Nck; and SHEP1, a R-Ras- and Rap-1A- development and how disregulation of these functions binding protein (reviewed by Bruckner and Klein, 1998; could promote tumorigenesis. Oncogene (2000) 19, Dodelet et al., 1999; Flanagan and Vanderhaeghen, 5614 ± 5619. 1998; Kalo and Pasquale, 1999). -
EPHB6 Augments Both Development and Drug Sensitivity of Triple- Negative Breast Cancer Tumours
Oncogene (2018) 37:4073–4093 https://doi.org/10.1038/s41388-018-0228-x ARTICLE EPHB6 augments both development and drug sensitivity of triple- negative breast cancer tumours 1 1,2 1 3 1 4 Behzad M. Toosi ● Amr El Zawily ● Luke Truitt ● Matthew Shannon ● Odette Allonby ● Mohan Babu ● 1 5 1 1 6 1 John DeCoteau ● Darrell Mousseau ● Mohsin Ali ● Tanya Freywald ● Amanda Gall ● Frederick S. Vizeacoumar ● 3 1 7 8 9 Morgan W. Kirzinger ● C. Ronald Geyer ● Deborah H. Anderson ● TaeHyung Kim ● Alana L. Welm ● 10 7 3 1 Peter Siegel ● Franco J. Vizeacoumar ● Anthony Kusalik ● Andrew Freywald Received: 17 May 2017 / Revised: 30 January 2018 / Accepted: 27 February 2018 / Published online: 27 April 2018 © The Author(s) 2018. This article is published with open access Abstract Triple-negative breast cancer (TNBC) tumours that lack expression of oestrogen, and progesterone receptors, and do not overexpress the HER2 receptor represent the most aggressive breast cancer subtype, which is characterised by the resistance to therapy in frequently relapsing tumours and a high rate of patient mortality. This is likely due to the resistance of slowly proliferating tumour-initiating cells (TICs), and understanding molecular mechanisms that control TICs behaviour is crucial fi 1234567890();,: for the development of effective therapeutic approaches. Here, we present our novel ndings, indicating that an intrinsically catalytically inactive member of the Eph group of receptor tyrosine kinases, EPHB6, partially suppresses the epithelial–mesenchymal transition in TNBC cells, while also promoting expansion of TICs. Our work reveals that EPHB6 interacts with the GRB2 adapter protein and that its effect on enhancing cell proliferation is mediated by the activation of the RAS-ERK pathway, which allows it to elevate the expression of the TIC-related transcription factor, OCT4.