Wind Inhomogeneities in WR Stars. II. Investigation of Emission-Line
Total Page:16
File Type:pdf, Size:1020Kb
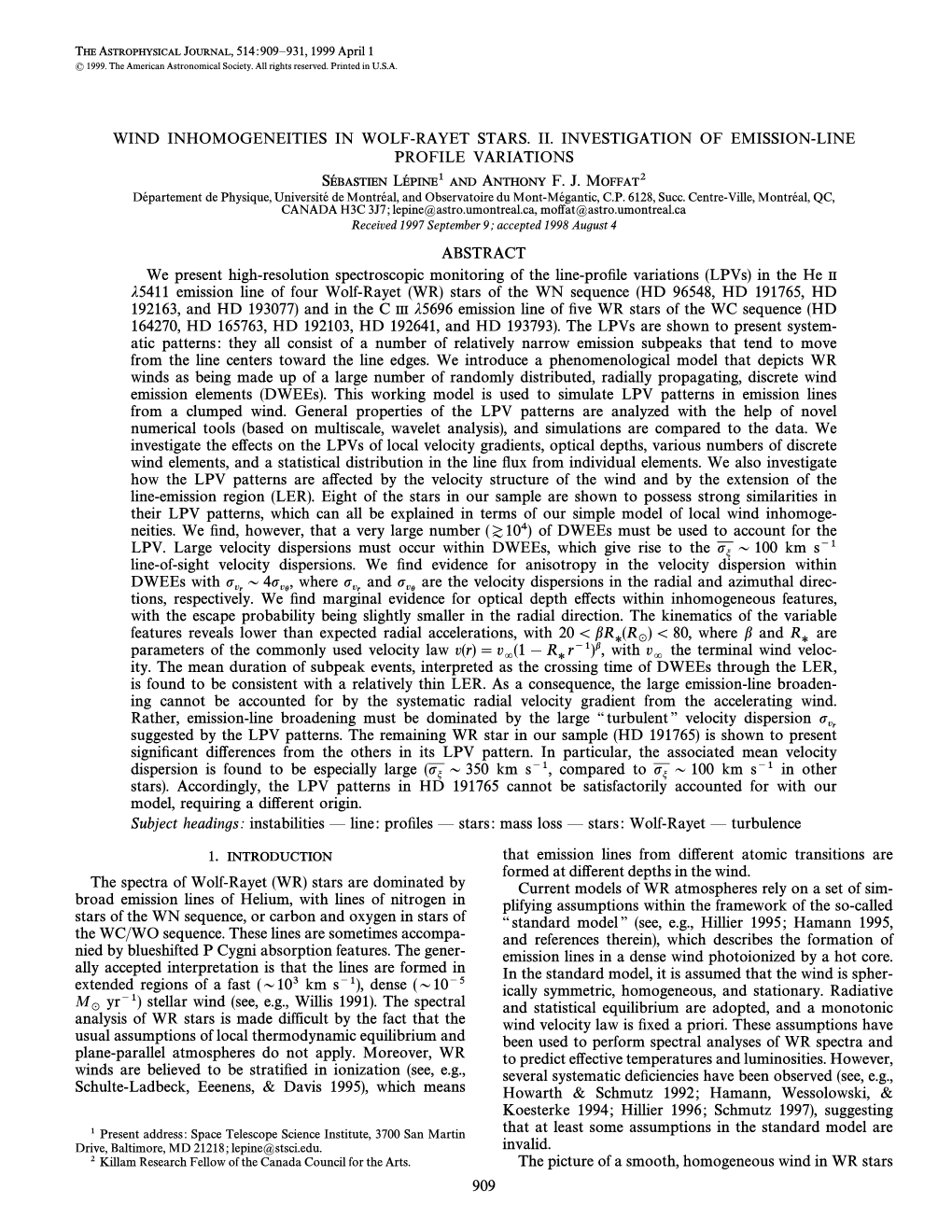
Load more
Recommended publications
-
Rotating Wolf-Rayet Stars in a Post RSG/LBV Phase an Evolutionary Channel Towards Long-Duration Grbs?
A&A 547, A83 (2012) Astronomy DOI: 10.1051/0004-6361/201118664 & c ESO 2012 Astrophysics Rotating Wolf-Rayet stars in a post RSG/LBV phase An evolutionary channel towards long-duration GRBs? G. Gräfener1,J.S.Vink1, T. J. Harries2, and N. Langer3 1 Armagh Observatory, College Hill, Armagh BT61 9DG, UK 2 School of Physics and Astronomy, University of Exeter, Stocker Rd, Exeter EX4 4QL, UK 3 Argelander-Institut für Astronomie der Universität Bonn, Auf dem Hügel 71, 53121 Bonn, Germany Received 16 December 2011 / Accepted 3 October 2012 ABSTRACT Context. Wolf-Rayet (WR) stars with fast rotating cores are thought to be the direct progenitors of long-duration gamma-ray bursts (LGRBs). A well accepted evolutionary channel towards LGRBs is chemically-homogeneous evolution at low metallicities, which completely avoids a red supergiant (RSG), or luminous blue variable (LBV) phase. On the other hand, strong absorption features with velocities of several hundred km s−1 have been found in some LGRB afterglow spectra (GRB 020813 and GRB 021004), which have been attributed to dense circumstellar (CS) material that has been ejected in a previous RSG or LBV phase, and is interacting with a fast WR-type stellar wind. Aims. Here we investigate the properties of Galactic WR stars and their environment to identify similar evolutionary channels that may lead to the formation of LGRBs. Methods. We compile available information on the spectropolarimetric properties of 29 WR stars, the presence of CS ejecta for 172 WR stars, and the CS velocities in the environment of 34 WR stars in the Galaxy. -
X-Ray Emission from Wolf-Rayet Stars
X-ray Emission from Wolf-Rayet Stars Steve Skinner1, Svet Zhekov2, Manuel Güdel3 Werner Schmutz4, Kimberly Sokal1 1CASA, Univ. of Colorado (USA) [email protected] 2Space Research Inst. (Bulgaria) and JILA/Univ. of Colorado (USA) 3ETH Zurich (Switzerland) 3PMOD (Switzerland) Abstract We present an overview of recent X-ray observations of Wolf-Rayet (WR) stars with XMM-Newton and Chandra. Observations of several WC-type (carbon-rich) WR stars without known companions have yielded only non-detections, implying they are either very feeble X-ray emitters or perhaps even X-ray quiet. In contrast, several apparently single WN2-6 stars have been detected, but data are sparse for later WN7-9 stars. Putatively single WN stars such as WR 134 have X-ray luminosities and spectra that are strikingly similar to some known WN + OB binaries such as WR 147, suggesting a similar emission mechanism. 1 X-rays from WR Stars: Overview 3 Single Nitrogen-rich WN Stars 4 Wolf-Rayet Binaries WR stars are the evolutionary descendants of massive O • Sensitive X-ray observations have now been obtained • High-resolution X-ray grating spectra have been ob- stars and are losing mass at very high rates. They are in ad- of several putatively single WN2-6 stars with XMM tained for a few binaries such as γ2 Vel (WC8 + vanced nuclear burning stages, approaching the end of their and Chandra. All but one were detected (Fig. 1). O7.5; Fig. 3) and WR 140 (WC7 + O4-5). CCD lives as supernovae. Strong X-rays have been detected from CCD spectra exist (Fig. -
A 2.4-12 Microns Spectrophotometric Study with ISO of Cygnus X-3 in Quiescence
1 Abstract. We present mid-infrared spectrophotometric results obtained with the ISO on the peculiar X-ray bi- nary Cygnus X-3 in quiescence, at orbital phases 0.83 to 1.04. The 2.4 - 12 µm continuum radiation observed with ISOPHOT-S can be explained by thermal free-free emis- sion in an expanding wind with, above 6.5 µm, a possi- ble additional black-body component with temperature T ∼ 250K and radius R ∼ 5000R⊙ at 10 kpc, likely due to thermal emission by circumstellar dust. The observed brightness and continuum spectrum closely match that of the Wolf-Rayet star WR 147, a WN8+B0.5 binary system, when rescaled at the same 10 kpc distance as Cygnus X- 3. A rough mass loss estimate assuming a WN wind gives −4 −1 ∼ 1.2 × 10 M⊙.yr . A line at ∼ 4.3 µm with a more than 4.3 σ detection level, and with a dereddened flux of 126 mJy, is interpreted as the expected He I 3p-3s line at 4.295 µm, a prominent line in the WR 147 spectrum. These results are consistent with a Wolf-Rayet-like com- panion to the compact object in Cyg X-3 of WN8 type, a later type than suggested by earlier works. Key words: binaries: close - stars: individual: Cyg X-3 - stars: Wolf-Rayet - stars: mass loss - infrared: stars arXiv:astro-ph/0207466v1 22 Jul 2002 A&A manuscript no. ASTRONOMY (will be inserted by hand later) AND Your thesaurus codes are: missing; you have not inserted them ASTROPHYSICS A 2.4 - 12 µm spectrophotometric study with ISO of CygnusX-3 in quiescence ⋆ Lydie Koch-Miramond1, P´eter Abrah´am´ 2,3, Ya¨el Fuchs1,4, Jean-Marc Bonnet-Bidaud1, and Arnaud Claret1 1 DAPNIA/Service d’Astrophysique, CEA-Saclay, 91191 Gif-sur-Yvette Cedex, France 2 Konkoly Observatory, P.O. -
198 6Apj. . .300. .37 9T the Astrophysical Journal, 300
9T .37 The Astrophysical Journal, 300:379-395,1986 January 1 © 1986. The American Astronomical Society. All rights reserved. Printed in U.S.A. .300. 6ApJ. 198 SPECTROSCOPIC STUDIES OF WOLF-RAYET STARS. III. THE WC SUBCLASS Ana V. Torres and Peter S. Conti1,2 Joint Institute for Laboratory Astrophysics, University of Colorado and National Bureau of Standards AND Philip Massey2 Kitt Peak National Observatory, National Optical Astronomy Observatories Received 1985 AprilS; accepted 1985 June 28 ABSTRACT We present spectrophotometric data for the major optical emission lines of 64 Galactic and 18 Large Magellanic Cloud (LMC) WC stars. Using line ratios of O v A5590, C m 25696, and C iv 25806 we quantify the subtype classification. A few Galactic stars are reclassified, and nearly all the LMC WC stars are found to be of type WC4. Thus there is even a greater discrepancy in the distribution of WC subtypes between the LMC and the Galaxy than previously assumed, since WC4 types in the Galaxy are rare. New measures of the line widths of C in 24650 are found to correlate nicely with the (revised) WC subtypes, although a few stars have lines too wide for their line ratios. Two of the most discrepant stars, WR 125 and WR 140, also show nonthermal radio emission and are strong X-ray sources. Terminal wind velocities are estimated from an excitation—line width relation. The terminal velocities range from 1000 km s_1 for the latest subtypes to 5000 km s "1 for the earliest types. Subject headings: galaxies: Magellanic Clouds — stars: stellar statistics— stars: winds — stars: Wolf-Rayet I. -
ŞAR Shao SPECIAL ISSUE 2013 CİLD 8 № 2 AZERBAIJANI ASTRONOMICAL JOURNAL
ISSN: 2078-4163 XÜSUSİ BURAXILIŞ ŞAR ShAO SPECIAL ISSUE 2013 CİLD 8 № 2 AZERBAIJANI ASTRONOMICAL JOURNAL ISSN: 2078-4163 Azәrbaycan Milli Elmlәr Akademiyası AZӘRBAYCAN ASTRONOMİYA JURNALI Cild 8 – № 2 – 2013 | XÜSUSİ BURAXILIŞ ŞAR - ShAO - ШАО - 60 Azerbaijan National Academy of Sciences Национальная Академия Наук Азербайджана AZERBAIJANI АСТРОНОМИЧЕСКИЙ ASTRONOMICAL ЖУРНАЛ JOURNAL АЗЕРБАЙДЖАНА Volume 8 – No 2 – 2013 Том 8 – № 2 – 2013 SPECIAL ISSUE СПЕЦИАЛЬНЫЙ ВЫПУСК Azәrbaycan Milli Elmlәr Akademiyasının “AZӘRBAYCAN ASTRONOMIYA JURNALI” Azәrbaycan Milli Elmlәr Akademiyası (AMEA) Rәyasәt Heyәtinin 28 aprel 2006-cı il tarixli 50-saylı Sәrәncamı ilә tәsis edilmişdir. Baş Redaktor: Ә.S. Quliyev Baş Redaktorun Müavini: E.S. Babayev Mәsul Katib: P.N. Şustarev REDAKSIYA HEYӘTİ: Cәlilov N.S. AMEA N.Tusi adına Şamaxı Astrofizika Rәsәdxanası Hüseynov R.Ә. Baki Dövlәt Universiteti İsmayılov N.Z. AMEA N.Tusi adına Şamaxı Astrofizika Rәsәdxanası Qasımov F. Q. AMEA Fizika İnsitutu Quluzadә C.M. Baki Dövlәt Universiteti Texniki redaktor: A.B. Әsgәrov İnternet sәhifәsi: http://www.shao.az/AAJ Ünvan: Azәrbaycan, Bakı, AZ-1001, İstiqlaliyyәt küç. 10, AMEA Rәyasәt Heyәti Jurnal AMEA N.Tusi adına Şamaxı Astrofizika Rәsәdxanasında (www.shao.az) nәşr olunur. Мәktublar üçün: ŞAR, Azәrbaycan, Bakı, AZ-1000, Mәrkәzi Poçtamt, a/q №153 e-mail: [email protected] tel.: (+99412) 439 82 48 faкs: (+99412) 497 52 68 2013 Azәrbaycan Milli Elmlәr Akademiyası. 2013 AMEA N.Tusi adına Şamaxı Astrofizika Rәsәdxanası. Bütün hüquqlar qorunmuşdur. Bakı – 2013 ____________________________________________________________________________________________________________ “Астрономический Журнал Азербайджана” Национальной Azerbaijani Astronomical Journal of the Azerbaijan National Академии Наук Азербайджана (НАНА). Academy of Sciences (ANAS) is founded in 28 Aprel 2006. Основан 28 апреля 2006 г. Web- адрес: http://www.shao.az/AAJ Online version: http://www.shao.az/AAJ Главный редактор: А.С.Гулиев Editor-in-Chief: A.S. -
The Results of the 2013 Pro-Am Wolf-Rayet Campaign E
Wolf-Rayet Stars W.-R. Hamann, A. Sander, H. Todt, eds. Potsdam: Univ.-Verlag, 2015 URL: http://nbn-resolving.de/urn:nbn:de:kobv:517-opus4-84268 The Results of the 2013 Pro-Am Wolf-Rayet Campaign E. J. Aldoretta1, N. St-Louis1, N. D. Richardson1, A. F. J. Moffat1, T. Eversberg2, G. M. Hill3 and the World-Wide WR Pro-Am Campaign Team4;5 1Universite´ de Montr´eal, Canada 2Schn¨orringenTelescope Science Institute, Germany 3W. M. Keck Observatory, United States 4VdS Section Spectroscopy, Germany 5Astronomical Ring for Access to Spectroscopy (ARAS), France Professional and amateur astronomers around the world contributed to a 4-month long cam- paign in 2013, mainly in spectroscopy but also in photometry, interferometry and polarimetry, to observe the first 3 Wolf-Rayet stars discovered: WR 134 (WN6b), WR 135 (WC8) and WR 137 (WC7pd+O9). Each of these stars are interesting in their own way, showing a variety of stellar wind structures. The spectroscopic data from this campaign were reduced and analyzed for WR 134 in order to better understand its behavior and long-term periodicity in the context of CIRs in the wind. We will be presenting the results of these spectroscopic data, which include the confirmation of the CIR variability and a time-coherency of 40 days (half-life of 20 days). ∼ ∼ 1 Motivation and Campaign the spectral lines (Morel et al. 1999), WR 135 is known to show large clumpy structures in its wind Overview (Lepine et al. 1996) and WR 137 is a long-period WR+O binary system with possible CIRs (Lef`evre Co-rotating interaction regions (CIRs) form in the et al. -
Spectropolarimetry As a Probe of Stellar Winds
Spectropolarimetry as a Probe of Stellar Winds Timothy James Harries Thesis submitted for the degree of Doctor of Philosophy in the Faculty of Science of the University of London. UCL Department of Physics & Astronomy U n iv e r s it y • C o l l e g e • L o n d o n February 1995 ProQuest Number: 10045605 All rights reserved INFORMATION TO ALL USERS The quality of this reproduction is dependent upon the quality of the copy submitted. In the unlikely event that the author did not send a complete manuscript and there are missing pages, these will be noted. Also, if material had to be removed, a note will indicate the deletion. uest. ProQuest 10045605 Published by ProQuest LLC(2016). Copyright of the Dissertation is held by the Author. All rights reserved. This work is protected against unauthorized copying under Title 17, United States Code. Microform Edition © ProQuest LLC. ProQuest LLC 789 East Eisenhower Parkway P.O. Box 1346 Ann Arbor, Ml 48106-1346 Abstract The use of spectropolarimetry as a diagnostic probe of stellar-wind structure is inves tigated by using high-quality observations and state-of-the-art analytical and numerical models. The winds of late-type giant stars are studied through the highly polarized 6830Â and 7088Â Raman-scattered emission hnes that are observed in many symbiotic systems. A spectropolarimetric survey of 28 symbiotic stars is presented. A Monte-Carlo code is developed in order to aid interpretation of the hnes and the parameter sensitivity of the Raman hne polarization spectrum is investigated. -
CURRICULUM VITAE: Dr Richard Ignace
CURRICULUM VITAE: Dr Richard Ignace Address: Department of Physics & Astronomy Office of Undergraduate Research College of Arts & Sciences Honors College EAST TENNESSEE STATE UNIVERSITY EAST TENNESSEE STATE UNIVERSITY Johnson City, TN 37614 Johnson City, TN 37614 Email: [email protected] [email protected] Web: faculty.etsu.edu/ignace www.etsu.edu/honors/ug research Phone/Fax: (423) 439-6904 / (423) 439-6905 (423) 439-6073 / (423) 439-6080 EDUCATION Ph.D. in Astronomy, University of Wisconsin 1996 M.S. in Physics, University of Wisconsin 1994 M.S. in Astronomy, University of Wisconsin 1993 B.S. in Astronomy, Indiana University 1991 POSITIONS HELD Aug 2016–present, Consultant, Tri-Alpha Energy Jan 2015–present, Director of Undergraduate Research Activities, East Tennessee State University Aug 2013–present, Full Professor: East Tennessee State University Aug 2007–Jul 2013, Associate Professor: East Tennessee State University Aug 2003–Jul 2007, Assistant Professor: East Tennessee State University Sep 2002–Jul 2003, Assistant Scientist: University of Wisconsin Aug 1999–Aug 2002, Visiting Assistant Professor: University of Iowa Nov 1996–Aug 1999, Postdoctoral Research Assistant: University of Glasgow SELECTED PROFESSIONAL ACTIVITIES Involved with service to discipline, institution, and community As Director of Undergraduate Research & Creative Activities, I administrate grant programs and activ- ities that support undergraduate scholarship, plus advocate for undergraduate research. Successful with publishing scholarly articles and competing for grant funding; author of the astron- omy textbook “Astro4U: An Introduction to the Science of the Cosmos,” of the popular astronomy book “Understanding the Universe,” and co-editor of the conference proceedings “The Nature and Evolution of Disks around Hot Stars” Principal organizer for STELLAR POLARIMETRY: FROM BIRTH TO DEATH, Jun 2011; and THE NATURE AND EVOLUTION OF DISKS AROUND HOT STARS, Jul 2004. -
Absence of Hot Gas Within the Wolft-Rayet Bubble Around WR16
Astronomy & Astrophysics manuscript no. ms c ESO 2018 April 30, 2018 Absence of hot gas within the Wolf-Rayet bubble around WR 16 J.A. Toal´aand M.A. Guerrero Instituto de Astrof´ısica de Andaluc´ıa, IAA-CSIC, Glorieta de la Astronom´ıa s/n, 18008 Granada, Spain; [email protected] Preprint online version: April 30, 2018 ABSTRACT We present the analysis of XMM-Newton archival observations towards the Wolf-Rayet (WR) bubble around WR 16. Despite the closed bubble morphology of this WR nebula, the XMM-Newton observations show no evidence of diffuse emission in its interior as in the similar WR bubbles NGC 6888 and S 308. We use the present observations to estimate a 3-σ upper limit to the X-ray luminosity in the 0.3–1.5 keV energy band equal to 7.4×1032 erg s−1 for the diffuse emission from the WR nebula, assuming a distance of 2.37 kpc. The WR nebula around WR 16 is the fourth observed by the current generation of X-ray satellites and the second not detected. We also examine FUSE spectra to search for nebular O vi absorption lines in the stellar continuum of WR 16. The present far-UV data and the lack of measurements of the dynamics of the optical WR bubble do not allow us to confirm the existence of a conductive layer of gas at T∼3×105 K between the cold nebular gas and the hot gas in its interior. The present observations result in an upper limit of −3 ne <0.6 cm on the electron density of the X-ray emitting material within the nebula. -
Interstellarum 89 | August/September 2013 Fokussiert
interstellarum 89 | August/September 2013 fokussiert Titelbild: IC 410 im Schmalbandfilter-Komposit, umgesetzt als Falschfarbenaufnahme nach der Hubble-Palette. Aufgenommen mit einem 300/1140-Newton und Korrektor, Moravian Instruments G2 8300 CCD-Kamera, 10×8min (Hα), 11×8min ([OIII]), 11×8min ([SII]). Lesen Sie dazu mehr ab Seite 42. Thomas Jäger INTERAKTIV Werden Sie Follower des neuen interstellarum Twitter-Dienstes! . Die gesamte Redaktion informiert t g Sie täglich über Neuigkeiten aus der (Amateur-)Astronomie. www.twitter.com/interstellarum ist untersa g BUCHTIPP reitun Liebe Leserinnen, liebe Leser, b ein Sternatlas der neuen Generation – das ist der neue »interstellarum Deep-Sky- interstellarum Erscheint Deep-Sky-Atlas Atlas«, der am 1. September erscheinen wird. Über zehn Jahre habe ich an diesem neuen am 1.9. Ronald Stoyan, Konzept gearbeitet: ein Kartenwerk, das Deep-Sky-Objekte nicht einfach als Symbole Stephan Schurig darstellt, sondern diese so gestaltet, dass helle Objekte auf einen Blick von schwachen zu unterscheiden sind; ein Kartenwerk, das so einfach und logisch aufgebaut ist, dass auch unter schwierigen Bedingungen die Orientierung leicht fällt; ein Kartenwerk, das mit vielen kleinen Details von visuellen Objektumrissen bis zur Filterempfehlung auf die Bedürfnisse des praktischen Deep-Sky-Beobachters eingeht. Ich möchte Sie herzlich einladen, sich unter www.deepskyatlas.de über unser größtes Projekt in der Geschichte des Oculum-Verlags zu informieren, und den Atlas auf der Astromesse in Villingen- Schwenningen am 14. September selbst in Augenschein zu nehmen (Seite 2). Die Astromesse AME wird auch der Höhepunkt in unserem großen Astrofotowett- bewerb »Astrofotograf des Jahres 2013« sein. Nachdem die Jury aus den Astrofotografen Bernhard Hubl, Johannes Schedler, Ranga Yogeshwar sowie Egon Döberl von unserem nur zu privaten Zwecken. -
Pennsylvania Science Olympiad Southeast Regional Tournament 2013 Astronomy C Division Exam March 4, 2013
PENNSYLVANIA SCIENCE OLYMPIAD SOUTHEAST REGIONAL TOURNAMENT 2013 ASTRONOMY C DIVISION EXAM MARCH 4, 2013 SCHOOL:________________________________________ TEAM NUMBER:_________________ INSTRUCTIONS: 1. Turn in all exam materials at the end of this event. Missing exam materials will result in immediate disqualification of the team in question. There is an exam packet as well as a blank answer sheet. 2. You may separate the exam pages. You may write in the exam. 3. Only the answers provided on the answer page will be considered. Do not write outside the designated spaces for each answer. 4. Include school name and school code number at the bottom of the answer sheet. Indicate the names of the participants legibly at the bottom of the answer sheet. Be prepared to display your wristband to the supervisor when asked. 5. Each question is worth one point. Tiebreaker questions are indicated with a (T#) in which the number indicates the order of consultation in the event of a tie. Tiebreaker questions count toward the overall raw score, and are only used as tiebreakers when there is a tie. In such cases, (T1) will be examined first, then (T2), and so on until the tie is broken. There are 12 tiebreakers. 6. When the time is up, the time is up. Continuing to write after the time is up risks immediate disqualification. 7. In the BONUS box on the answer sheet, name the gentleman depicted on the cover for a bonus point. 8. As per the 2013 Division C Rules Manual, each team is permitted to bring “either two laptop computers OR two 3-ring binders of any size, or one binder and one laptop” and programmable calculators. -
A Consistent Spectral Model of WR 136 and Its Associated Bubble NGC 6888 J
Wolf-Rayet Stars W.-R. Hamann, A. Sander, H. Todt, eds. Potsdam: Univ.-Verlag, 2015 URL: http://nbn-resolving.de/urn:nbn:de:kobv:517-opus4-84268 A consistent spectral model of WR 136 and its associated bubble NGC 6888 J. Reyes-P´erez1, C. Morisset1, M. Pen~a1 & A. Mesa-Delgado2 1Instituto de Astronom´ıa,Universidad Nacional Aut´onomade M´exico, M´exico 2Instituto de Astrof´ısica, Facultad de F´ısica, Pontificia Universidad Cat´olica de Chile We analyse whether a stellar atmosphere model computed with the code CMFGEN provides an optimal description of the stellar observations of WR 136 and simultaneously reproduces the nebular observations of NGC 6888, such as the ionization degree, which is modelled with the pyCloudy code. All the observational material available (far and near UV and optical spectra) were used to constrain such models. We found that the stellar temperature T , at τ = 20, can be in a range between 70 000 and 110 000 K, but when using the nebula as an additional∗ restriction, we found that the stellar models with T 70 000 K represent the best solution for both, the star and the nebula. ∗ ∼ 1 Introduction hydrostatic radius of the star R (at optical depth τ equal to 20) and the chemical composition.∗ NGC 6888 is an emission nebula associated with the As the modelled spectral features do not respond Wolf-Rayet star WR 136 with spectral type WN6(h), linearly to the input parameters, we calculated a and both have been already studied in several wave- grid of models in order to find the more appropri- length ranges from X-rays to radio emission, but all ated models which reproduce most of the observed the previous works focus only on the modelling of the spectral signatures.