Molecular Mechanisms of Ampa and Kainate Receptor Gating and Its Implication in Synaptic Transmission
Total Page:16
File Type:pdf, Size:1020Kb
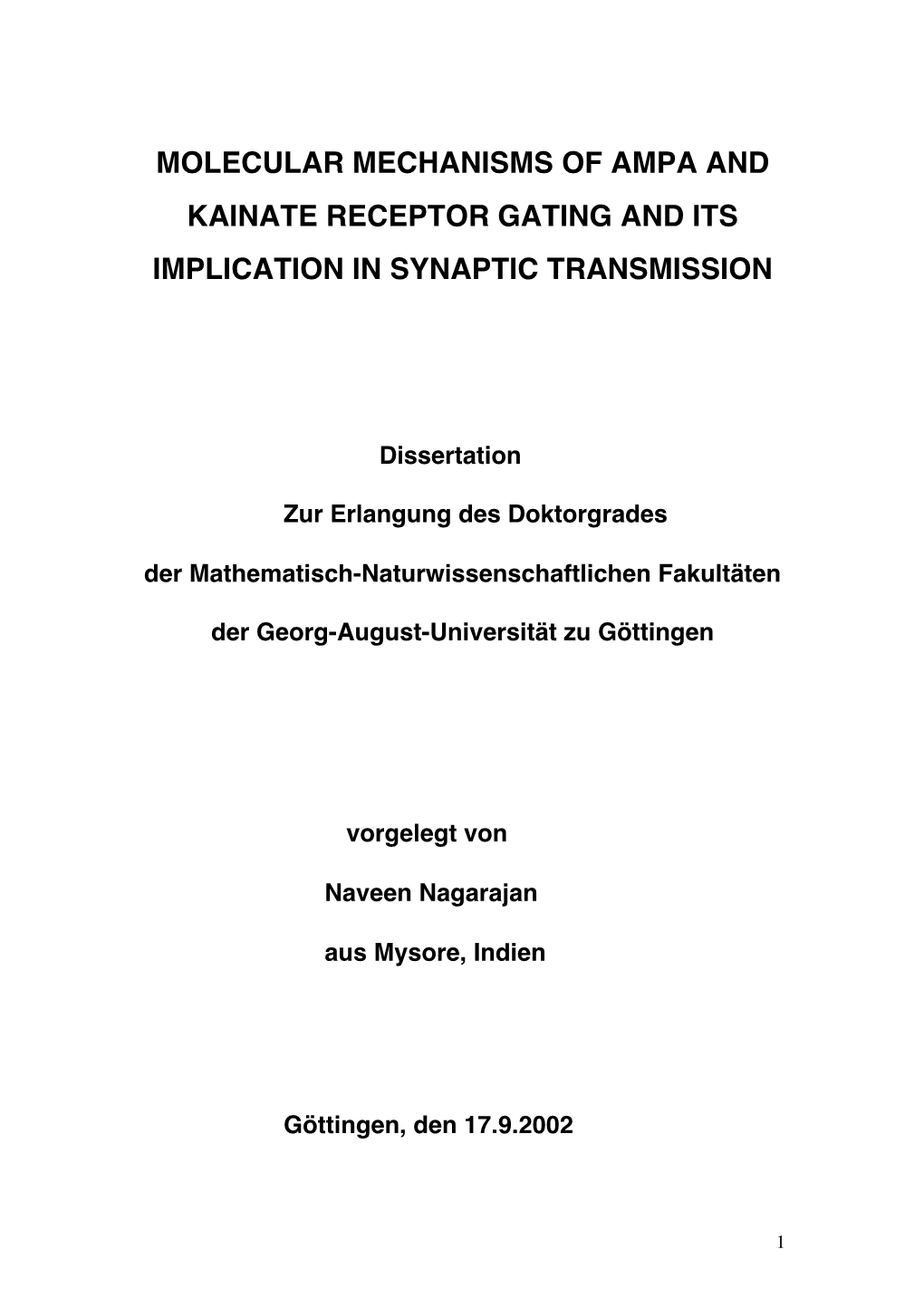
Load more
Recommended publications
-
Effects of Chronic Systemic Low-Impact Ampakine Treatment On
Biomedicine & Pharmacotherapy 105 (2018) 540–544 Contents lists available at ScienceDirect Biomedicine & Pharmacotherapy journal homepage: www.elsevier.com/locate/biopha Effects of chronic systemic low-impact ampakine treatment on neurotrophin T expression in rat brain ⁎ Daniel P. Radin , Steven Johnson, Richard Purcell, Arnold S. Lippa RespireRx Pharmaceuticals, Inc., 126 Valley Road, Glen Rock, NJ, 07452, United States ARTICLE INFO ABSTRACT Keywords: Neurotrophin dysregulation has been implicated in a large number of neurodegenerative and neuropsychiatric Ampakine diseases. Unfortunately, neurotrophins cannot cross the blood brain barrier thus, novel means of up regulating BDNF their expression are greatly needed. It has been demonstrated previously that neurotrophins are up regulated in Cognitive enhancement response to increases in brain activity. Therefore, molecules that act as cognitive enhancers may provide a LTP clinical means of up regulating neurotrophin expression. Ampakines are a class of molecules that act as positive Neurotrophin allosteric modulators of AMPA-type glutamate receptors. Currently, they are being developed to prevent opioid- NGF induced respiratory depression without sacrificing the analgesic properties of the opioids. In addition, these molecules increase neuronal activity and have been shown to restore age-related deficits in LTP in aged rats. In the current study, we examined whether two different ampakines could increase levels of BDNF and NGF at doses that are active in behavioral measures of cognition. Results demonstrate that ampakines CX516 and CX691 induce differential increases in neurotrophins across several brain regions. Notable increases in NGF were ob- served in the dentate gyrus and piriform cortex while notable BDNF increases were observed in basolateral and lateral nuclei of the amygdala. -
(19) United States (12) Patent Application Publication (10) Pub
US 20130289061A1 (19) United States (12) Patent Application Publication (10) Pub. No.: US 2013/0289061 A1 Bhide et al. (43) Pub. Date: Oct. 31, 2013 (54) METHODS AND COMPOSITIONS TO Publication Classi?cation PREVENT ADDICTION (51) Int. Cl. (71) Applicant: The General Hospital Corporation, A61K 31/485 (2006-01) Boston’ MA (Us) A61K 31/4458 (2006.01) (52) U.S. Cl. (72) Inventors: Pradeep G. Bhide; Peabody, MA (US); CPC """"" " A61K31/485 (201301); ‘4161223011? Jmm‘“ Zhu’ Ansm’ MA. (Us); USPC ......... .. 514/282; 514/317; 514/654; 514/618; Thomas J. Spencer; Carhsle; MA (US); 514/279 Joseph Biederman; Brookline; MA (Us) (57) ABSTRACT Disclosed herein is a method of reducing or preventing the development of aversion to a CNS stimulant in a subject (21) App1_ NO_; 13/924,815 comprising; administering a therapeutic amount of the neu rological stimulant and administering an antagonist of the kappa opioid receptor; to thereby reduce or prevent the devel - . opment of aversion to the CNS stimulant in the subject. Also (22) Flled' Jun‘ 24’ 2013 disclosed is a method of reducing or preventing the develop ment of addiction to a CNS stimulant in a subj ect; comprising; _ _ administering the CNS stimulant and administering a mu Related U‘s‘ Apphcatlon Data opioid receptor antagonist to thereby reduce or prevent the (63) Continuation of application NO 13/389,959, ?led on development of addiction to the CNS stimulant in the subject. Apt 27’ 2012’ ?led as application NO_ PCT/US2010/ Also disclosed are pharmaceutical compositions comprising 045486 on Aug' 13 2010' a central nervous system stimulant and an opioid receptor ’ antagonist. -
The Role of Diallyl Thiosulfinate Associated with Nuciferine And
Cai_Stesura Seveso 27/03/18 09:29 Pagina 59 DOI: 10.4081/aiua.2018.1.59 ORIGINAL PAPER The role of diallyl thiosulfinate associated with nuciferine and diosgenin in the treatment of premature ejaculation: A pilot study Tommaso Cai 1, Andrea Cocci 2, Giamartino Cito 2, Bruno Giammusso 3, Alessandro Zucchi 4, Francesco Chiancone 5, Maurizio Carrino 5, Francesco Mastroeni 6, Francesco Comerci 7, Giorgio Franco 8, Alessandro Palmieri 9 1 Department of Urology, Santa Chiara Regional Hospital, Trento, Italy; 2 Department of Urology, University of Florence, Florence, Italy; 3 Department of Andrology, Policlinico Morgagni, Catania, Italy; 4 Department of Urology, University of Perugia, Perugia, Italy; 5 Department of Urology, Cardarelli Hospital, Naples, Italy; 6 Department of Urology, Azienda Ospedaliera Papardo, Messina, Italy 7 Urologist, Bologna, Italy; 8 Department of Urology, Sapienza University of Rome, Rome, Italy; 9 Department of Urology, University of Naples, Federico II, Naples, Italy. Summary Objective: To assess the efficacy and safety with prevalence rates of 20-30%, probably affecting of an association of diallyl thiosulfinate with every man at some point in his life (2, 3). It may result nuciferine and diosgenin in the treatment of a group of patients in unsatisfactory sexual intercourse for both partners, suffering from premature ejaculation (PE), primary or second- decreasing sexual self-confidence and self-esteem and ary to erectile dysfunction (ED). resulting in an overall reduction of quality of life (QoL) Materials and methods: From July 2015 to October 2016, 143 (4). Lifelong PE occurs within 30-60 seconds after vagi- patients (mean age 25.3; range 18-39) affected by PE complet- ed the study and were finally analyzed in this phase I study. -
Neuroenhancement in Healthy Adults, Part I: Pharmaceutical
l Rese ca arc ni h li & C f B o i o l e Journal of a t h n Fond et al., J Clinic Res Bioeth 2015, 6:2 r i c u s o J DOI: 10.4172/2155-9627.1000213 ISSN: 2155-9627 Clinical Research & Bioethics Review Article Open Access Neuroenhancement in Healthy Adults, Part I: Pharmaceutical Cognitive Enhancement: A Systematic Review Fond G1,2*, Micoulaud-Franchi JA3, Macgregor A2, Richieri R3,4, Miot S5,6, Lopez R2, Abbar M7, Lancon C3 and Repantis D8 1Université Paris Est-Créteil, Psychiatry and Addiction Pole University Hospitals Henri Mondor, Inserm U955, Eq 15 Psychiatric Genetics, DHU Pe-psy, FondaMental Foundation, Scientific Cooperation Foundation Mental Health, National Network of Schizophrenia Expert Centers, F-94000, France 2Inserm 1061, University Psychiatry Service, University of Montpellier 1, CHU Montpellier F-34000, France 3POLE Academic Psychiatry, CHU Sainte-Marguerite, F-13274 Marseille, Cedex 09, France 4 Public Health Laboratory, Faculty of Medicine, EA 3279, F-13385 Marseille, Cedex 05, France 5Inserm U1061, Idiopathic Hypersomnia Narcolepsy National Reference Centre, Unit of sleep disorders, University of Montpellier 1, CHU Montpellier F-34000, Paris, France 6Inserm U952, CNRS UMR 7224, Pierre and Marie Curie University, F-75000, Paris, France 7CHU Carémeau, University of Nîmes, Nîmes, F-31000, France 8Department of Psychiatry, Charité-Universitätsmedizin Berlin, Campus Benjamin Franklin, Eschenallee 3, 14050 Berlin, Germany *Corresponding author: Dr. Guillaume Fond, Pole de Psychiatrie, Hôpital A. Chenevier, 40 rue de Mesly, Créteil F-94010, France, Tel: (33)178682372; Fax: (33)178682381; E-mail: [email protected] Received date: January 06, 2015, Accepted date: February 23, 2015, Published date: February 28, 2015 Copyright: © 2015 Fond G, et al. -
Are AMPA Receptor Positive Allosteric Modulators Potential Pharmacotherapeutics for Addiction?
Pharmaceuticals 2014, 7, 29-45; doi:10.3390/ph7010029 OPEN ACCESS pharmaceuticals ISSN 1424-8247 www.mdpi.com/journal/pharmaceuticals Review Are AMPA Receptor Positive Allosteric Modulators Potential Pharmacotherapeutics for Addiction? Lucas R. Watterson 1,* and M. Foster Olive 1,2 1 Department of Psychology, Behavioral Neuroscience Area, Arizona State University, Tempe, AZ 85287, USA 2 Interdisciplinary Graduate Program in Neuroscience, Arizona State University, Tempe, AZ 85287, USA * Author to whom correspondence should be addressed; E-Mail:[email protected]; Tel.: +1-480-965-2573. Received: 28 October 2013; in revised form: 13 December 2013 / Accepted: 24 December 2013 / Published: 30 December 2013 Abstract: Positive allosteric modulators (PAMs) of α-amino-3-hydroxy-5-methyl-4- isoxazolepropionic acid (AMPA) receptors are a diverse class of compounds that increase fast excitatory transmission in the brain. AMPA PAMs have been shown to facilitate long-term potentiation, strengthen communication between various cortical and subcortical regions, and some of these compounds increase the production and release of brain-derived neurotrophic factor (BDNF) in an activity-dependent manner. Through these mechanisms, AMPA PAMs have shown promise as broad spectrum pharmacotherapeutics in preclinical and clinical studies for various neurodegenerative and psychiatric disorders. In recent years, a small collection of preclinical animal studies has also shown that AMPA PAMs may have potential as pharmacotherapeutic adjuncts to extinction-based or cue-exposure therapies for the treatment of drug addiction. The present paper will review this preclinical literature, discuss novel data collected in our laboratory, and recommend future research directions for the possible development of AMPA PAMs as anti-addiction medications. -
Preferred Drug List 4-Tier
Preferred Drug List 4-Tier 21NVHPN13628 Four-Tier Base Drug Benefit Guide Introduction As a member of a health plan that includes outpatient prescription drug coverage, you have access to a wide range of effective and affordable medications. The health plan utilizes a Preferred Drug List (PDL) (also known as a drug formulary) as a tool to guide providers to prescribe clinically sound yet cost-effective drugs. This list was established to give you access to the prescription drugs you need at a reasonable cost. Your out- of-pocket prescription cost is lower when you use preferred medications. Please refer to your Prescription Drug Benefit Rider or Evidence of Coverage for specific pharmacy benefit information. The PDL is a list of FDA-approved generic and brand name medications recommended for use by your health plan. The list is developed and maintained by a Pharmacy and Therapeutics (P&T) Committee comprised of actively practicing primary care and specialty physicians, pharmacists and other healthcare professionals. Patient needs, scientific data, drug effectiveness, availability of drug alternatives currently on the PDL and cost are all considerations in selecting "preferred" medications. Due to the number of drugs on the market and the continuous introduction of new drugs, the PDL is a dynamic and routinely updated document screened regularly to ensure that it remains a clinically sound tool for our providers. Reading the Drug Benefit Guide Benefits for Covered Drugs obtained at a Designated Plan Pharmacy are payable according to the applicable benefit tiers described below, subject to your obtaining any required Prior Authorization or meeting any applicable Step Therapy requirement. -
Enhancement of Ampakine-Induced
(19) & (11) EP 1 715 863 B1 (12) EUROPEAN PATENT SPECIFICATION (45) Date of publication and mention (51) Int Cl.: of the grant of the patent: A61K 31/445 (2006.01) A61K 31/40 (2006.01) 09.05.2012 Bulletin 2012/19 A61K 31/4525 (2006.01) A61K 45/06 (2006.01) (21) Application number: 05712023.0 (86) International application number: PCT/US2005/002372 (22) Date of filing: 26.01.2005 (87) International publication number: WO 2005/072345 (11.08.2005 Gazette 2005/32) (54) ENHANCEMENT OF AMPAKINE-INDUCED FACILITATION OF SYNAPTIC RESPONSES BY CHOLINESTERASE INHIBITORS VERBESSERUNG DER DURCH AMPAKINE INDUZIERTEN ERLEICHTERUNG VON SYNAPTISCHEN REAKTIONEN DURCH CHOLINESTERASE-INHIBITOREN RENFORCEMENT DE LA FACILITATION INDUITE PAR AMPAKINE DES REPONSES SYNAPTIQUES PAR LES INHIBITEURS DE LA CHOLINESTERASE (84) Designated Contracting States: (74) Representative: Baldock, Sharon Claire AT BE BG CH CY CZ DE DK EE ES FI FR GB GR Boult Wade Tennant HU IE IS IT LI LT LU MC NL PL PT RO SE SI SK TR Verulam Gardens 70 Gray’s Inn Road (30) Priority: 26.01.2004 US 539422 P London WC1X 8BT (GB) (43) Date of publication of application: (56) References cited: 02.11.2006 Bulletin 2006/44 EP-A- 1 203 584 US-A- 5 747 492 US-B2- 6 689 795 (73) Proprietors: • CORTEX PHARMACEUTICALS, INC. • JOHNSON STEVEN A ET AL: "Randomized, Irvine, CA 92618 (US) double-blind, placebo-controlled international • The Regents of The University of California clinical trial of the Ampakine CX516 in elderly Oakland, CA 94607-5200 (US) participants with mild cognitive impairment. -
Mechanisms of Addiction
Mechanisms of Addiction Prof David Nutt, FMedSci Centre for Neuropsychopharmacology, Division of Brain Sciences, Burlington Danes Building, Imperial College London, London W12 0NN, UK. [email protected] www.tocris.com What is addiction? Although the term addiction is controversial because it can be stigmatizing and divisive, we use it here as a useful shorthand for the processes by which people become dependent on the use of drugs, or activities such as gambling, to the point where it distorts and even takes over their lives. The key feature of drug addiction is the inability to stop using the drug despite clear evidence of harm; the drug becomes progressively more important, dominating life at the expense of family, friends and work. Not all those who use drugs become addicted and factors that predispose to addiction include the class of drug, family history of addiction and propensity to withdrawal reactions and craving. Using drugs to deal with psychiatric problems is particularly likely to lead to addiction. Brain Circuits Associated with Addiction Classes of Addictive Drugs Products available from Tocris Stimulants Stimulants are drugs that act by releasing dopamine and, D Cocaine, (D)-Amphetamine sulfate, 3 Morphine, Mephedrone, in some cases, blocking its reuptake. This class includes Threo-methylphenidate D cocaine (and crack), amphetamine and its derivatives such 1,5 MAO as metamphetamine (crystal meth) and methylphenidate, + ATP (R)-(-)-Deprenyl, Moclobemide DA and some cathinones, such as mephedrone. Their actions DAT PFC AC are dependent on speed of brain penetration, with smoking cAMP PKA GBR 12909, Tetrabenazine, Reserpine, MAO VMAT and iv injection the most addictive routes, but are largely – (±)-MDMA CREB Dopamine D and D ACG unaffected by dopamine receptor antagonists. -
Ampakine CX1942 Attenuates Opioid-Induced Respiratory Depression and Corrects the Hypoxaemic Effects of Etorphine in Immobilized Goats (Capra Hircus)
Ampakine CX1942 attenuates opioid-induced respiratory depression and corrects the hypoxaemic effects of etorphine in immobilized goats (Capra hircus) Anna J Haw*, Leith CR Meyer*,†, John J Greer‡ & Andrea Fuller*,† * Brain Function Research Group, Faculty of Health Sciences, School of Physiology, University of the Witwatersrand, Parktown, South Africa †Department of Paraclinical Sciences, Faculty of Veterinary Science, University of Pretoria, Onderstepoort, South Africa ‡Department of Physiology, Faculty of Medicine and Dentistry, University of Alberta, Edmonton, AB, Canada Correspondence: Anna J Haw, Brain Function Research Group, Faculty of Health Sciences, School of Physiology, University of the Witwatersrand, 7 York Road, Parktown 2193, South Africa. E-mail: [email protected] Abstract Objectives: To determine whether CX1942 reverses respiratory depression in etorphine- immobilized goats, and to compare its effects with those of doxapram hydrochloride. Study design: A prospective, crossover experimental trial conducted at 1753 m.a.s.l. Animals: Eight adult female Boer goats (Capra hircus) with a mean ± standard deviation mass of 27.1 ± 1.6 kg. Methods: Following immobilization with 0.1 mg kg−1 etorphine, goats received one of doxapram, CX1942 or sterile water intravenously, in random order in three trials. Respiratory rate, ventilation and tidal volume were measured continuously. Arterial blood samples for the determination of PaO2, PaCO2, pH and SaO2 were taken 2 minutes before and then at 5 minute intervals after drug administration for 25 minutes. Results: Doxapram corrected etorphine-induced respiratory depression but also led to arousal and hyperventilation at 2 minutes after its administration, as indicated by the low −1 PaCO2 (27.8 ± 4.5 mmHg) and ventilation of 5.32 ± 5.24 L minute above pre-immobilization values. -
Glutamate Receptor Ion Channels: Structure, Regulation, and Function Stephen Traynelis, Emory University Lonnie P
Glutamate Receptor Ion Channels: Structure, Regulation, and Function Stephen Traynelis, Emory University Lonnie P. Wollmuth, SUNY Stony Brook Chris J. McBain, Eunice Kennedy Shriver Natl Inst Child Hlth & Hum Frank S. Menniti, CyclicM LLC Katie M. Vance, Emory University Kevin K. Ogden, Emory University Kasper B. Hansen, Emory University Hongjie Yuan, Emory University Scott J. Myers, Emory University Raymond Dingledine, Emory University Journal Title: Pharmacological Reviews Volume: Volume 62, Number 3 Publisher: American Society for Pharmacology and Experimental Therapeutics (ASPET) | 2010-09-01, Pages 405-496 Type of Work: Article | Final Publisher PDF Publisher DOI: 10.1124/pr.109.002451 Permanent URL: https://pid.emory.edu/ark:/25593/tws3d Final published version: http://dx.doi.org/10.1124/pr.109.002451 Copyright information: U.S. Government work not protected by U.S. copyright Accessed September 23, 2021 7:10 AM EDT Glutamate Receptor Ion Channels: Structure, Regulation, and Function Stephen Traynelis, Emory University Lonnie P. Wollmuth, Stony Brook University Chris J. McBain, Eunice Kennedy Shriver National Institute of Child Health and Human Development Frank S. Menniti, cyclicM LLC Katie M. Vance, Emory University Kevin K. Ogden, Emory University Kasper B. Hansen, Emory University Hongjie Yuan, Emory University Scott J. Myers, Emory University Raymond J Dingledine, Emory University Journal Title: Pharmacological Reviews Volume: Volume 62, Number 3 Publisher: American Society for Pharmacology and Experimental Therapeutics (ASPET) | 2010-09, Pages 405-496 Type of Work: Article | Final Publisher PDF Publisher DOI: 10.1124/pr.109.002451 Permanent URL: http://pid.emory.edu/ark:/25593/f867k Final published version: http://pharmrev.aspetjournals.org/content/62/3/405 Copyright information: U.S. -
The Glutamate Receptor Ion Channels
0031-6997/99/5101-0007$03.00/0 PHARMACOLOGICAL REVIEWS Vol. 51, No. 1 Copyright © 1999 by The American Society for Pharmacology and Experimental Therapeutics Printed in U.S.A. The Glutamate Receptor Ion Channels RAYMOND DINGLEDINE,1 KARIN BORGES, DEREK BOWIE, AND STEPHEN F. TRAYNELIS Department of Pharmacology, Emory University School of Medicine, Atlanta, Georgia This paper is available online at http://www.pharmrev.org I. Introduction ............................................................................. 8 II. Gene families ............................................................................ 9 III. Receptor structure ...................................................................... 10 A. Transmembrane topology ............................................................. 10 B. Subunit stoichiometry ................................................................ 10 C. Ligand-binding sites located in a hinged clamshell-like gorge............................. 13 IV. RNA modifications that promote molecular diversity ....................................... 15 A. Alternative splicing .................................................................. 15 B. Editing of AMPA and kainate receptors ................................................ 17 V. Post-translational modifications .......................................................... 18 A. Phosphorylation of AMPA and kainate receptors ........................................ 18 B. Serine/threonine phosphorylation of NMDA receptors .................................. -
Kynurenines and the Endocannabinoid System in Schizophrenia: Common Points and Potential Interactions
View metadata, citation and similar papers at core.ac.uk brought to you by CORE provided by SZTE Publicatio Repozitórium - SZTE - Repository of Publications molecules Review Kynurenines and the Endocannabinoid System in Schizophrenia: Common Points and Potential Interactions 1, 2,3, 4 1 Ferenc Zádor y,Gábor Nagy-Grócz y, Gabriella Kekesi , Szabolcs Dvorácskó , Edina Sz ˝ucs 1,5, Csaba Tömböly 1, Gyongyi Horvath 4,Sándor Benyhe 1 and László Vécsei 3,6,* 1 Institute of Biochemistry, Biological Research Center, Temesvári krt. 62., H-6726 Szeged, Hungary; [email protected] (F.Z.); [email protected] (S.D.); [email protected] (E.S.); [email protected] (C.T.); [email protected] (S.B.) 2 Faculty of Health Sciences and Social Studies, University of Szeged, Temesvári krt. 31., H-6726 Szeged, Hungary; [email protected] 3 Department of Neurology, Faculty of Medicine, Albert Szent-Györgyi Clinical Center, University of Szeged, Semmelweis u. 6., H-6725 Szeged, Hungary 4 Department of Physiology, Faculty of Medicine, University of Szeged, Dóm tér 10., H-6720 Szeged, Hungary; [email protected] (G.K.); [email protected] (G.H.) 5 Doctoral School of Theoretical Medicine, Faculty of Medicine, University of Szeged, Dóm tér 10., H-6720 Szeged, Hungary 6 Interdisciplinary Excellence Center, Department of Neurology, University of Szeged, Semmelweis u. 6., H-6725 Szeged, Hungary * Correspondence: [email protected]; Tel.: +36-62-545-351 These authors contributed equally to the work. y Academic Editor: Raffaele Capasso Received: 30 August 2019; Accepted: 14 October 2019; Published: 15 October 2019 Abstract: Schizophrenia, which affects around 1% of the world’s population, has been described as a complex set of symptoms triggered by multiple factors.