Loss of Dominant Caterpillar Genera in a Protected Tropical Forest Danielle M
Total Page:16
File Type:pdf, Size:1020Kb
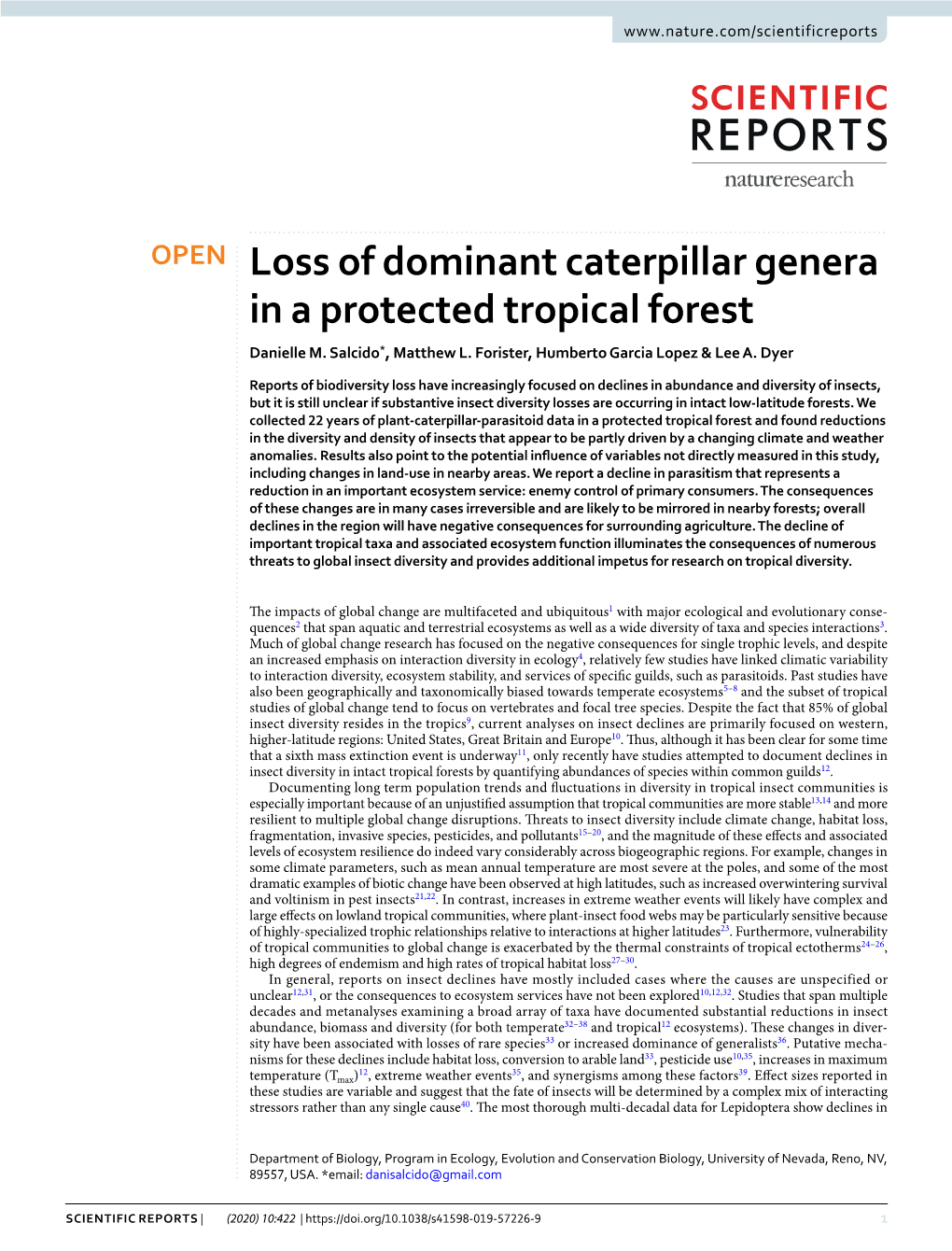
Load more
Recommended publications
-
Lepidoptera of North America 5
Lepidoptera of North America 5. Contributions to the Knowledge of Southern West Virginia Lepidoptera Contributions of the C.P. Gillette Museum of Arthropod Diversity Colorado State University Lepidoptera of North America 5. Contributions to the Knowledge of Southern West Virginia Lepidoptera by Valerio Albu, 1411 E. Sweetbriar Drive Fresno, CA 93720 and Eric Metzler, 1241 Kildale Square North Columbus, OH 43229 April 30, 2004 Contributions of the C.P. Gillette Museum of Arthropod Diversity Colorado State University Cover illustration: Blueberry Sphinx (Paonias astylus (Drury)], an eastern endemic. Photo by Valeriu Albu. ISBN 1084-8819 This publication and others in the series may be ordered from the C.P. Gillette Museum of Arthropod Diversity, Department of Bioagricultural Sciences and Pest Management Colorado State University, Fort Collins, CO 80523 Abstract A list of 1531 species ofLepidoptera is presented, collected over 15 years (1988 to 2002), in eleven southern West Virginia counties. A variety of collecting methods was used, including netting, light attracting, light trapping and pheromone trapping. The specimens were identified by the currently available pictorial sources and determination keys. Many were also sent to specialists for confirmation or identification. The majority of the data was from Kanawha County, reflecting the area of more intensive sampling effort by the senior author. This imbalance of data between Kanawha County and other counties should even out with further sampling of the area. Key Words: Appalachian Mountains, -
Reading the Complex Skipper Butterfly Fauna of One Tropical Place
Reading the Complex Skipper Butterfly Fauna of One Tropical Place Daniel H. Janzen1*, Winnie Hallwachs1, John M. Burns2, Mehrdad Hajibabaei3, Claudia Bertrand3, Paul D. N. Hebert3 1 Department of Biology, University of Pennsylvania, Philadelphia, Pennsylvania, United States of America, 2 Department of Entomology, National Museum of Natural History, Smithsonian Institution, Washington, D.C., United States of America, 3 Department of Integrative Biology, Biodiversity Institute of Ontario, University of Guelph, Guelph, Canada Abstract Background: An intense, 30-year, ongoing biodiversity inventory of Lepidoptera, together with their food plants and parasitoids, is centered on the rearing of wild-caught caterpillars in the 120,000 terrestrial hectares of dry, rain, and cloud forest of Area de Conservacion Guanacaste (ACG) in northwestern Costa Rica. Since 2003, DNA barcoding of all species has aided their identification and discovery. We summarize the process and results for a large set of the species of two speciose subfamilies of ACG skipper butterflies (Hesperiidae) and emphasize the effectiveness of barcoding these species (which are often difficult and time-consuming to identify). Methodology/Principal Findings: Adults are DNA barcoded by the Biodiversity Institute of Ontario, Guelph, Canada; and they are identified by correlating the resulting COI barcode information with more traditional information such as food plant, facies, genitalia, microlocation within ACG, caterpillar traits, etc. This process has found about 303 morphologically defined species of eudamine and pyrgine Hesperiidae breeding in ACG (about 25% of the ACG butterfly fauna) and another 44 units indicated by distinct barcodes (n = 9,094), which may be additional species and therefore may represent as much as a 13% increase. -
Conservation and Management of Eastern Big-Eared Bats a Symposium
Conservation and Management of Eastern Big-eared Bats A Symposium y Edited b Susan C. Loeb, Michael J. Lacki, and Darren A. Miller U.S. Department of Agriculture Forest Service Southern Research Station General Technical Report SRS-145 DISCLAIMER The use of trade or firm names in this publication is for reader information and does not imply endorsement by the U.S. Department of Agriculture of any product or service. Papers published in these proceedings were submitted by authors in electronic media. Some editing was done to ensure a consistent format. Authors are responsible for content and accuracy of their individual papers and the quality of illustrative materials. Cover photos: Large photo: Craig W. Stihler; small left photo: Joseph S. Johnson; small middle photo: Craig W. Stihler; small right photo: Matthew J. Clement. December 2011 Southern Research Station 200 W.T. Weaver Blvd. Asheville, NC 28804 Conservation and Management of Eastern Big-eared Bats: A Symposium Athens, Georgia March 9–10, 2010 Edited by: Susan C. Loeb U.S Department of Agriculture Forest Service Southern Research Station Michael J. Lacki University of Kentucky Darren A. Miller Weyerhaeuser NR Company Sponsored by: Forest Service Bat Conservation International National Council for Air and Stream Improvement (NCASI) Warnell School of Forestry and Natural Resources Offield Family Foundation ContEntS Preface . v Conservation and Management of Eastern Big-Eared Bats: An Introduction . 1 Susan C. Loeb, Michael J. Lacki, and Darren A. Miller Distribution and Status of Eastern Big-eared Bats (Corynorhinus Spp .) . 13 Mylea L. Bayless, Mary Kay Clark, Richard C. Stark, Barbara S. -
Butterflies (Lepidoptera: Papilionoidea) in a Coastal Plain Area in the State of Paraná, Brazil
62 TROP. LEPID. RES., 26(2): 62-67, 2016 LEVISKI ET AL.: Butterflies in Paraná Butterflies (Lepidoptera: Papilionoidea) in a coastal plain area in the state of Paraná, Brazil Gabriela Lourenço Leviski¹*, Luziany Queiroz-Santos¹, Ricardo Russo Siewert¹, Lucy Mila Garcia Salik¹, Mirna Martins Casagrande¹ and Olaf Hermann Hendrik Mielke¹ ¹ Laboratório de Estudos de Lepidoptera Neotropical, Departamento de Zoologia, Universidade Federal do Paraná, Caixa Postal 19.020, 81.531-980, Curitiba, Paraná, Brazil Corresponding author: E-mail: [email protected]٭ Abstract: The coastal plain environments of southern Brazil are neglected and poorly represented in Conservation Units. In view of the importance of sampling these areas, the present study conducted the first butterfly inventory of a coastal area in the state of Paraná. Samples were taken in the Floresta Estadual do Palmito, from February 2014 through January 2015, using insect nets and traps for fruit-feeding butterfly species. A total of 200 species were recorded, in the families Hesperiidae (77), Nymphalidae (73), Riodinidae (20), Lycaenidae (19), Pieridae (7) and Papilionidae (4). Particularly notable records included the rare and vulnerable Pseudotinea hemis (Schaus, 1927), representing the lowest elevation record for this species, and Temenis huebneri korallion Fruhstorfer, 1912, a new record for Paraná. These results reinforce the need to direct sampling efforts to poorly inventoried areas, to increase knowledge of the distribution and occurrence patterns of butterflies in Brazil. Key words: Atlantic Forest, Biodiversity, conservation, inventory, species richness. INTRODUCTION the importance of inventories to knowledge of the fauna and its conservation, the present study inventoried the species of Faunal inventories are important for providing knowledge butterflies of the Floresta Estadual do Palmito. -
(Lepidoptera) of the Tuxtlas Mts., Veracruz, Mexico, Revisited: Species-Richness and Habitat Disturbance
29(1-2):105-133,Journal of Research 1990(91) on the Lepidoptera 29(1-2):105-133, 1990(91) 105 The Butterflies (Lepidoptera) of the Tuxtlas Mts., Veracruz, Mexico, Revisited: Species-Richness and Habitat Disturbance. Robert A. Raguso Dept. of Biology, Yale University, New Haven, CT 06511 USA.* Jorge Llorente-Bousquets Museo de Zoologia, Facultad de Ciencias, Universidad Nacional Autonoma de Mexico, Apartado Postal 70-399 Mexico D.F., CP 04510 Abstract. Checklists of the butterflies (Lepidoptera) collected in two rainforest study sites in the Tuxtlas Mts., Veracruz, Mexico are presented. A total of 182 species of butterflies were recorded at Laguna Encantada, near San Andres Tuxtla, and 212 species were recorded from the nearby Estacion de Biologia Tropical “Los Tuxtlas” (EBITROLOTU). We collected 33 species not included in G. Ross’ (1975–77) faunistic treatment of the region, 12 of which are new species records for the Tuxtlas. We present a list of the skipper butterflies (Hesperioidea) of the Tuxtlas, including a state record for the giant skipper, Agathymus rethon. At both study sites, we observed seasonal patterns in species abundance during periods of reduced precipitation. Our data indicate an apparent increase in butterfly species-richness in the Tuxtlas over the last 25 years. This increase reflects more efficient sampling due to advances in lepidopteran ecology and improved collecting methods, as well as the effects of habitat disturbance. A comparison between the butterfly faunas of the two rainforest sites revealed that a higher percentage of weedy, cosmopolitan species were present at Laguna Encantada, the smaller, more disturbed site. We anticipate further changes in butterfly species-richness and faunal composition as the mosaic of habitats in the Tuxtlas continue to be modified. -
Butterflies (Lepidoptera: Hesperioidea and Papilionoidea) of Porto Mauá, Upper Paraná Atlantic Forest Ecoregion, Rio Grande Do Sul State, Brazil
Biota Neotropica 14(2): e20130006, 2014 www.scielo.br/bn inventory Butterflies (Lepidoptera: Hesperioidea and Papilionoidea) of Porto Maua´, Upper Parana´ Atlantic Forest Ecoregion, Rio Grande do Sul State, Brazil Sabrina Campos Thiele1, Oscar Milcharek2,Fa´bio Luis dos Santos3 & Lucas Augusto Kaminski4,5 1PPG-Biologia Animal, Departamento de Zoologia, Universidade Federal do Rio Grande do Sul – UFRGS, Av. Bento Gonc¸alves, 9500, CEP 91501-970, Porto Alegre, Rio Grande do Sul, Brazil. 2Rua Caxias, 1376, Centro, CEP 98900-000, Santa Rosa, Rio Grande do Sul, Brazil. 3PPG-Entomologia, Departamento de Zoologia, Universidade Federal do Parana´–– UFPR, C.P. 19020, CEP 81531-980, Curitiba, Parana´, Brazil. 4Departamento de Biologia Animal, Universidade Estadual de Campinas – UNICAMP, C.P. 6109, CEP 13.083-970, Campinas, Sa˜o Paulo, Brazil. 5Corresponding author: Lucas Augusto Kaminski, e-mail: [email protected] THIELE, S.C., MILCHAREK, O., SANTOS, F.L. & KAMINSKI, L.A. Butterflies (Lepidoptera: Hesperioidea and Papilionoidea) of Porto Maua´, Upper Parana´ Atlantic Forest Ecoregion, Rio Grande do Sul State, Brazil. Biota Neotropica. 14(2): e20130006. http://dx.doi.org/10.1590/1676-06032014000613 Abstract: This paper presents a list of species of butterflies (Lepidoptera: Hesperioidea and Papilionoidea) sampled in Porto Maua´ municipality (27634’S, 28640’W), Rio Grande do Sul State, Brazil. Sampling was carried out monthly between March 2008 and March 2009. After 204 net-hours of sampling effort, a total of 1,993 individuals from 253 species were recorded. With a single additional expedition, eight new species were added, reaching a total of 261 species recorded in the region of Porto Maua´. -
Insect Egg Size and Shape Evolve with Ecology but Not Developmental Rate Samuel H
ARTICLE https://doi.org/10.1038/s41586-019-1302-4 Insect egg size and shape evolve with ecology but not developmental rate Samuel H. Church1,4*, Seth Donoughe1,3,4, Bruno A. S. de Medeiros1 & Cassandra G. Extavour1,2* Over the course of evolution, organism size has diversified markedly. Changes in size are thought to have occurred because of developmental, morphological and/or ecological pressures. To perform phylogenetic tests of the potential effects of these pressures, here we generated a dataset of more than ten thousand descriptions of insect eggs, and combined these with genetic and life-history datasets. We show that, across eight orders of magnitude of variation in egg volume, the relationship between size and shape itself evolves, such that previously predicted global patterns of scaling do not adequately explain the diversity in egg shapes. We show that egg size is not correlated with developmental rate and that, for many insects, egg size is not correlated with adult body size. Instead, we find that the evolution of parasitoidism and aquatic oviposition help to explain the diversification in the size and shape of insect eggs. Our study suggests that where eggs are laid, rather than universal allometric constants, underlies the evolution of insect egg size and shape. Size is a fundamental factor in many biological processes. The size of an 526 families and every currently described extant hexapod order24 organism may affect interactions both with other organisms and with (Fig. 1a and Supplementary Fig. 1). We combined this dataset with the environment1,2, it scales with features of morphology and physi- backbone hexapod phylogenies25,26 that we enriched to include taxa ology3, and larger animals often have higher fitness4. -
Psyche 87:121
LIFE HISTORY OF D YSODIA SICA (LEPIDOPTERA: THYRIDIDAE) IN PANAMA' Smithsonian Tropical Research Institute P.O. Box 2072, Balboa, Panama Thyrididae is a widespread tropical and subtropical family of moths, generally thought to be related to the Pyralidae, from which they differ in a number of ways (see Whalley, 1971). Possibly the group is not monophyletic, but so little is known concerning the immature stages, that few conclusions can be reached regarding the relationships among the four subfamilies. Dysodia (Thyrididae: Pachythyrinae) is a pantropical genus found on all continents except Australia (Whalley, 1971). Aside from scattered foodplant records for several species, little is known about the immature stages of any of the approximately thirty species. The present paper describes the egg, larvae, pupa, and larval behavior of Dysodia sica Druce (Figure 1) reared on Barro Colorado Island (BCI), Panama, during May through August 1977 and 1980. Seven other species of Dysodia are listed for BCI by Forbes (1942). Adults of Dysodia spp. fly during early evening, and are seen frequently at lights on BCI. At rest, the wings are horizontal to the substrate, with the fore wings almost completely covering the hind wings (Figure 2). This resting posture, triangular in outline, is also common among many pyralid moths. When alert or about to fly, the fore wings are drawn forwards, exposing the hind wings, and bringing the markings of the fore and hind wings into alignment (Figure 3). The two "windows" on each hind wing show just behind the posterior margin of the fore wings. The fore wings are curved longitudinally so as to be somewhat concave above. -
The Microlepidoptera Section 1 Limacodidae Through Cossidae
The University of Maine DigitalCommons@UMaine Technical Bulletins Maine Agricultural and Forest Experiment Station 8-1-1983 TB109: A List of the Lepidoptera of Maine--Part 2: The icrM olepidoptera Section 1 Limacodidae Through Cossidae Auburn E. Brower Follow this and additional works at: https://digitalcommons.library.umaine.edu/aes_techbulletin Part of the Entomology Commons Recommended Citation Brower, A.E. 1983. A list of the Lepidoptera of Maine--Part 2: The icrM olepidoptera Section 1 Limacodidae through Cossidae. Maine Agricultural Experiment Station Technical Bulletin 109. This Article is brought to you for free and open access by DigitalCommons@UMaine. It has been accepted for inclusion in Technical Bulletins by an authorized administrator of DigitalCommons@UMaine. For more information, please contact [email protected]. A LIST OF THE LEPIDOPTERA OF MAINE Part 2 THE MICROLEPIDOPTERA Section I LIMACODIDAE THROUGH COSSIDAE Auburn E. Brower A JOINT PUBLICATION OF THE MAINE DEPARTMENT OF CONSERVATION Maine Forest Service Division of Entomology, Augusta, Maine and the DEPARTMENT OF ENTOMOLOGY. ORONO Maine Agricultural Experiment Station August 198! Representatives of the Diverse Groups of Included Microlepidoptera 1 Slug moth 2 Pyralid moth 3 Argyrid moth 4 Plume moth 5 Bell moth 6 Cosmopterygid moth 7 Gelechiid moth 8 Ethmiid moth 9 Gracilariid moth 10 Glyphipterygid moth 11 Aegeriid moth Inquiries regarding this bulletin may be sent to: Dr. Auburn E. Brower 8 Hospital Street Augusta, Maine 04330 A LIST OF THE LEPIDOPTERA OF MAINE Part 2 THE MICROLEPIDOPTERA Section I LIMACODIDAE THROUGH COSSIDAE Auburn E. Brower A JOINT PUBLICATION OF THE MAINE DEPARTMENT OF CONSERVATION Maine Forest Service Division of Entomology, Augusta, Maine and the DEPARTMENT OF ENTOMOLOGY. -
A List of Cuban Lepidoptera (Arthropoda: Insecta)
TERMS OF USE This pdf is provided by Magnolia Press for private/research use. Commercial sale or deposition in a public library or website is prohibited. Zootaxa 3384: 1–59 (2012) ISSN 1175-5326 (print edition) www.mapress.com/zootaxa/ Article ZOOTAXA Copyright © 2012 · Magnolia Press ISSN 1175-5334 (online edition) A list of Cuban Lepidoptera (Arthropoda: Insecta) RAYNER NÚÑEZ AGUILA1,3 & ALEJANDRO BARRO CAÑAMERO2 1División de Colecciones Zoológicas y Sistemática, Instituto de Ecología y Sistemática, Carretera de Varona km 3. 5, Capdevila, Boyeros, Ciudad de La Habana, Cuba. CP 11900. Habana 19 2Facultad de Biología, Universidad de La Habana, 25 esq. J, Vedado, Plaza de La Revolución, La Habana, Cuba. 3Corresponding author. E-mail: rayner@ecologia. cu Table of contents Abstract . 1 Introduction . 1 Materials and methods. 2 Results and discussion . 2 List of the Lepidoptera of Cuba . 4 Notes . 48 Acknowledgments . 51 References . 51 Appendix . 56 Abstract A total of 1557 species belonging to 56 families of the order Lepidoptera is listed from Cuba, along with the source of each record. Additional literature references treating Cuban Lepidoptera are also provided. The list is based primarily on literature records, although some collections were examined: the Instituto de Ecología y Sistemática collection, Havana, Cuba; the Museo Felipe Poey collection, University of Havana; the Fernando de Zayas private collection, Havana; and the United States National Museum collection, Smithsonian Institution, Washington DC. One family, Schreckensteinidae, and 113 species constitute new records to the Cuban fauna. The following nomenclatural changes are proposed: Paucivena hoffmanni (Koehler 1939) (Psychidae), new comb., and Gonodontodes chionosticta Hampson 1913 (Erebidae), syn. -
Proceedingscalif09cali
' BINDING LIST DEC 1 5 )9l1 Digitized by tine Internet Arciiive in 2009 with funding from Ontario Council of University Libraries http://www.archive.org/details/proceedingscalif09cali PROCEEDINGS California Academy of Sciences FOURTH SERIES Vol. IX 1919 o^n y ,% " PRINTED FROM THE ^• r JOHN W. HENDRIE PUBLICATION ENDOWMENT SAN FRANCISCO Published by the Academy 1919 r m COMMITTEE ON PUBLICATION George C. Edwards, Chairman C. E. Grunsky Barton Warren Evermann, Editor if CONTENTS OF VOLUME IX Plates 1-20 page Title-page ] Contents '" Notes on West American Chitons— II 1 By S. Stillman Berry (Published June 16, 1919) Life-Zone Indicators in California 37 By Harvey Monroe Hall and Joseph Grinnell (Published June 16, 1919) Notes on Mammals collected principally in Washington and California between the Years 1853 and 1874 by Dr. James Graham Cooper. ... 69 By Walter P. Taylor (Published July 12, 1919) Climatic Relations of the Tertiary and Quaternary Faunas of the California Region 123 By James Perrin Smith (Published July 12, 1919) Contribution to the Optics of the Microscope 175 By C. W. Woodworth (Published July 12, 1919) The Gopher-Snakes of Western North America 197 By John Van Denburgh and Joseph R. Slevin (Published August 26, 1919) New Oregon Diptera 221 By F. R. Cole and A. L. Lovett (Published August 26, 1919) Key to the North American Species of the Dipterous Genus Medeterus, with Descriptions of New Species 257 By Millard C. Van Duzee (Published August 26, 1919.1 Description of a New Fossil Fish from Japan 271 By David Starr Jordan (Published October 22, 1919) Notes on the Avifauna of the Inner Coast Range of California 273 By Joseph Mailliard (Published November 25, 1919) New Species of Flies (Diptera) from California 297 By J. -
Fernandez Triana Et Al Prasmodon
JHR $$: Review@–@ (2014) of the Neotropical genus Prasmodon (Hymenoptera, Braconidae, Microgastrinae)... 1 doi: 10.3897/JHR.@@.6748 RESEARCH ARTICLE www.pensoft.net/journals/jhr Review of the Neotropical genus Prasmodon (Hymenoptera, Braconidae, Microgastrinae), with emphasis on species from Area de Conservación Guanacaste, northwestern Costa Rica Jose L. Fernandez-Triana1,2,†, James B. Whitfield3,‡, Alex M. Smith4,§, Winnie Hallwachs5,|, Daniel H. Janzen5,¶ 1 Department of Integrative Biology and the Biodiversity Institute of Ontario, University of Guelph, Guelph, ON N1G 2W1 Canada 2 Canadian National Collection of Insects, 960 Carling Ave., Ottawa, ON K1A 0C6 Canada 3 Department of Entomology, University of Illinois, Urbana, IL 61801 USA 4 Unité d’Entomologie fonctionnelle et évolutive, Gembloux Agro-Bio Tech, Université de Liège, B-1030 Gembloux, Belgique; and Département d’entomologie, IRSNB, Rue Vautier 29, 1000 Bruxelles, Belgique 5 Department of Biology, University of Pennsylvania, Philadelphia, PA 19104-6018 USA † urn:lsid:zoobank.org:author: ‡ urn:lsid:zoobank.org:author: § urn:lsid:zoobank.org:author: | urn:lsid:zoobank.org:author: ¶ urn:lsid:zoobank.org:author: Corresponding author: Jose Fernandez-Triana ([email protected]) Academic editor: G. Broad | Received 4 December 2013 | Accepted 14 March2014 | Published @@ @@@@ 2014 urn:lsid:zoobank.org:pub: Citation: Fernández-Triana JL, Whitfield JB, Smith MA, Braet Y, Hallwachs W, Janzen DH (2014)Review of the Neotropical genus Prasmodon (Hymenoptera, Braconidae, Microgastrinae),