Substrate Specificity of Glycine Oxidase and Protein Interaction Specificity of the Neuronal Cell Adhesion Molecule TAG-1
Total Page:16
File Type:pdf, Size:1020Kb
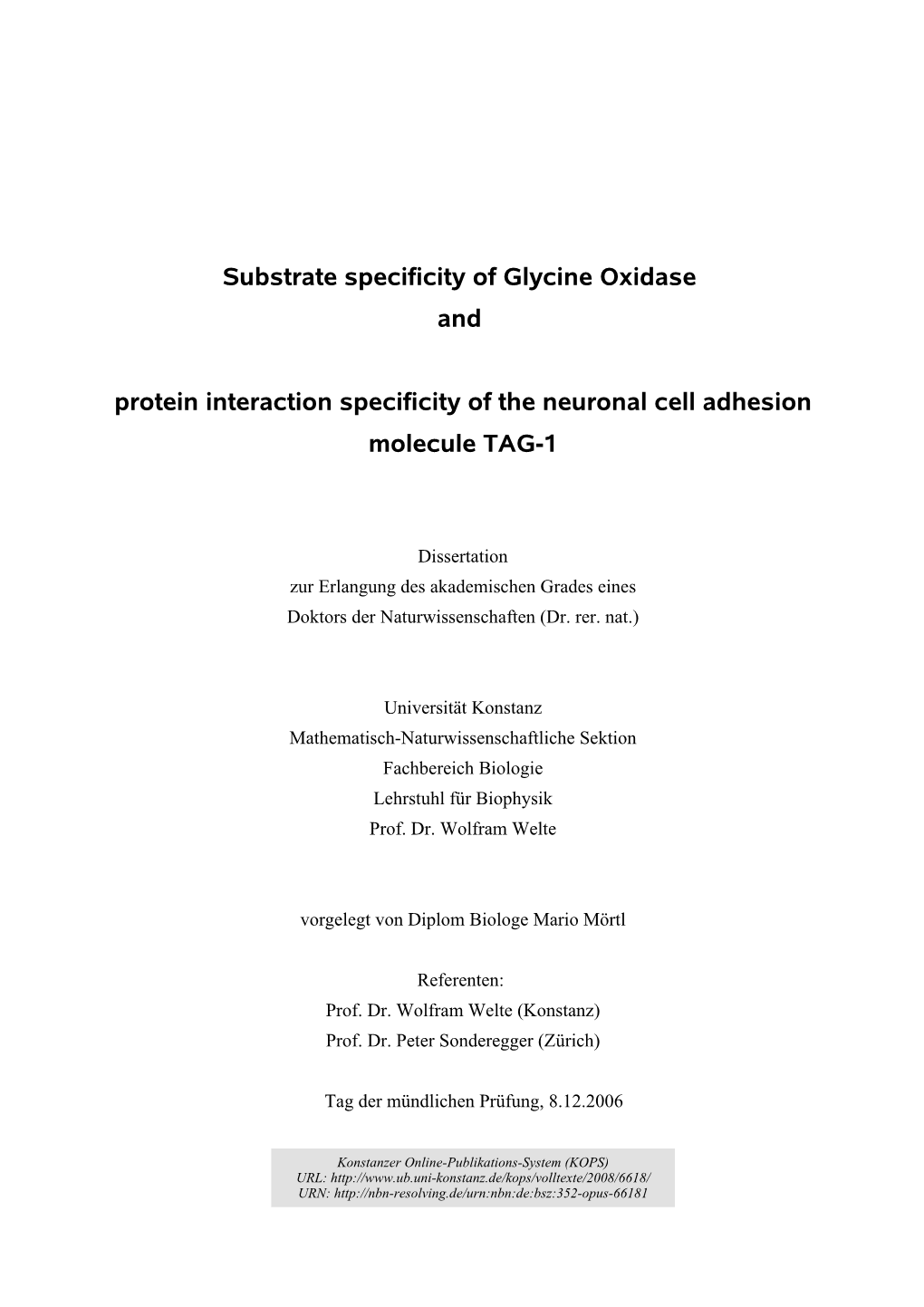
Load more
Recommended publications
-
Distribution in Different Organisms of Amino Acid Oxidases with FAD Or a Quinone As Cofactor and Their Role As Antimicrobial Proteins in Marine Bacteria
Supplementary Materials: Distribution in Different Organisms of Amino Acid Oxidases with FAD or a Quinone As Cofactor and Their Role as Antimicrobial Proteins in Marine Bacteria Jonatan C. Campillo‐Brocal, Patricia Lucas‐Elío and Antonio Sanchez‐Amat * Table S1. Amino acid oxidases (AAOs) from microbial sources. Marine microorganisms are shown in bold. * cofactor and/or activity attributed for high similarity with LodA. ND, not determined. NA, not accessible. LAAOs, L‐amino acid oxidases. DAAOs, D‐amino acid oxidases. LASPOs, L‐aspartate oxidases. CTQ, cysteine tryptophilquinone. Microorganism Oligomeric Structure/Mass Accession Activity (Main Substrate) Cofactor Various Reference (Enzyme Name) Molecular Number AAOs with Quinone Cofactor (LodA‐Like Proteins) Marinomonas Homotetramer (80.9 × 4 kDa). Antimicrobial. Biofilms mediterranea MMB‐1 L‐Lysine ε‐oxidase (L‐Lys) CTQ Crystal structure solved, PDB AAY33849 [1,2] dispersion. Extracellular (LodA) ID: 2YMW Marinomonas Other substrates: Gly ethyl mediterranea MMB‐1 Glycine oxidase (Gly) CTQ 76.2 kDa ADZ90918 [3,4] ester (GoxA) Pseudoalteromonas Antimicrobial. Biofilms L‐Lysine ε‐oxidase (L‐Lys) * CTQ 110 kDa Q7X0I8 [5,6] tunicata D2 (AlpP) dispersion Chromobacterium Antimicrobial. Biofilms ND ND ND AAQ60932 [6] violaceum dispersion Antimicrobial. Biofilms Caulobacter crescentus ND ND ND NP_419374 [6] dispersion Pseudoalteromonas flavipulchra JG1 * L‐Lysine ε‐oxidase (* L‐Lys) * CTQ 77 kDa Antimicrobial. pI = 4.6 AFB71049 [7] (PfaP) Broad spectrum oxidase Antimicrobial. pI = 9.4. It Pseudoalteromonas (L‐Lys > L‐Met > L‐Glu > L‐Leu > L‐ ND 60 kDa contains a 9‐residues peptide NA [8] flavipulchra C2 Gln > L‐Tyr > L‐Phe) similar to AlpP S1 Table S1. Cont. Broad spectrum oxidase Pseudoalteromonas (L‐Met > L‐Gln > L‐Leu > L‐Phe > L‐ ND Oligomer (110 kDa) Antimicrobial NA [9] luteoviolacea Glu > L‐Trp) Antimicrobial. -
Amino Acid Catabolism by Ribbed Mussel (Modiolus Demissus) Gill Tissue: Studies on Isolated Mitochondria and the L-Amino Acid Oxidase James M
Iowa State University Capstones, Theses and Retrospective Theses and Dissertations Dissertations 1983 Amino acid catabolism by ribbed mussel (Modiolus demissus) gill tissue: studies on isolated mitochondria and the L-amino acid oxidase James M. Burcham Iowa State University Follow this and additional works at: https://lib.dr.iastate.edu/rtd Part of the Agriculture Commons, Aquaculture and Fisheries Commons, and the Zoology Commons Recommended Citation Burcham, James M., "Amino acid catabolism by ribbed mussel (Modiolus demissus) gill tissue: studies on isolated mitochondria and the L-amino acid oxidase " (1983). Retrospective Theses and Dissertations. 8456. https://lib.dr.iastate.edu/rtd/8456 This Dissertation is brought to you for free and open access by the Iowa State University Capstones, Theses and Dissertations at Iowa State University Digital Repository. It has been accepted for inclusion in Retrospective Theses and Dissertations by an authorized administrator of Iowa State University Digital Repository. For more information, please contact [email protected]. INFORMATION TO USERS This reproduction was made from a copy of a document sent to us for microfilming. While the most advanced technology has been used to photograph and reproduce this document, the quality of the reproduction is heavily dependent upon the quality of the material submitted. The following explanation of techniques is provided to help clarify markings or notations which may appear on this reproduction. 1.The sign or "target" for pages apparently lacking from the document photographed is "Missing Page(s)". If it was possible to obtain the missing page(s) or section, they are spliced into the film along with adjacent pages. -
Curriculum Vitae Et Studiorum Di Loredano
Pubblicazioni del Dr. Gianluca Molla Pubblicazioni su Libri: 1. Pollegioni L, Campaner S, Molla G, Martegani E, Pilone MS (1996) Cloning and expression in E.coli of D-amino acid oxidase gene from Rhodotorula gracilis in Flavins and Flavoproteins (Stevenson K.J., ed.), 227-230 2. Pollegioni L, Umhau S, Molla G, Harris CM, Ghisla S, Pilone MS (1999) Reaction mechanism of flavin dehydrogenation by D-amino acid oxidase in Flavins and Flavoproteins (Ghisla S., ed.), 551-558. ISBN: 3-00-005128-7 3. Umhau S, Diederichs K, Welte W, Ghisla S, Pollegioni L, Molla G, Porrini D, Pilone MS (1999) Very high resolution crystal structure of D-amino acid oxidase. Insights into the reaction mechanism and mode of ligand binding in Flavins and Flavoproteins (Ghisla S., ed.), 567-570. ISBN: 3-00-005128-7 4. Molla G, Harris CM, Boselli A, Sacchi S, Pilone MS, Pollegioni L (1999) Structure and function of Rhodotorula gracilis D-amino acid oxidase. 1. Site-directed mutagenesis of tyrosines 223 and 238 in Flavins and Flavoproteins (Ghisla S., ed.), 559-562. ISBN: 3-00- 005128-7 5. Job V, Harris CM, Porrini D, Molla G, Vegezzi C, Motteran L, Ghisla S, Pollegioni L Pilone MS (1999) Structure and function of Rhodotorula gracilis D-amino acid oxidase. 2. Site-directed mutagenesis of arginine 285 and pH effects in Flavins and Flavoproteins (Ghisla S., ed.), 563-566. ISBN: 3-00-005128-7 6. Rizzi S, Molla G, Fantinato S, Pollegioni L (1999) Regulation of D-amino acid oxidase expression in the obligatory aerobic yeast Rhodotorula gracilis in Flavins and Flavoproteins (Ghisla S., ed.), 595-598. -
Mechanistic Insights Into the Radical S-Adenosyl L-Methionine Enzyme MFTC
University of Denver Digital Commons @ DU Electronic Theses and Dissertations Graduate Studies 1-1-2017 Mechanistic Insights into the Radical S-Adenosyl L-Methionine Enzyme MFTC Bulat Khaliullin University of Denver Follow this and additional works at: https://digitalcommons.du.edu/etd Part of the Biochemistry, Biophysics, and Structural Biology Commons, and the Chemistry Commons Recommended Citation Khaliullin, Bulat, "Mechanistic Insights into the Radical S-Adenosyl L-Methionine Enzyme MFTC" (2017). Electronic Theses and Dissertations. 1300. https://digitalcommons.du.edu/etd/1300 This Thesis is brought to you for free and open access by the Graduate Studies at Digital Commons @ DU. It has been accepted for inclusion in Electronic Theses and Dissertations by an authorized administrator of Digital Commons @ DU. For more information, please contact [email protected],[email protected]. MECHANISTIC INSIGHTS INTO THE RADICAL S-ADENOSYL L-METHIONINE ENZYME MFTC __________ A Thesis Presented to the Faculty of Natural Sciences and Mathematics University of Denver __________ In Partial Fulfillment of the Requirements for the Degree Master of Science __________ by Bulat Khaliullin June 2017 Advisor: John A. Latham ©Copyright by Bulat Khaliullin 2017 All Rights Reserved Author: Bulat Khaliullin Title: MECHANISTIC INSIGHTS INTO THE RADICAL S-ADENOSYL L- METHIONINE ENZYME MFTC Advisor: John A. Latham Degree Date: June 2017 Abstract Mycofactocin is a putative peptide-derived redox cofactor in Mycobacterium family. Its putative biosynthetic pathway is encoded by the operon mftABCDEF. The initial step of this pathway is a posttranslational modification of a peptide precursor MftA, which is catalyzed by MftC enzyme. This modification only occurs in the presence of chaperone MftB. -
A Novel Pathway to Produce Butanol and Isobutanol in Saccharomyces
Branduardi et al. Biotechnology for Biofuels 2013, 6:68 http://www.biotechnologyforbiofuels.com/content/6/1/68 RESEARCH Open Access A novel pathway to produce butanol and isobutanol in Saccharomyces cerevisiae Paola Branduardi1*†, Valeria Longo1†, Nadia Maria Berterame1, Giorgia Rossi2 and Danilo Porro1 Abstract Background: The sustainable production of biofuels remains one of the major issues of the upcoming years. Among the number of most desirable molecules to be produced, butanol and isobutanol deserve a prominent place. They have superior liquid-fuel features in respect to ethanol. Particularly, butanol has similar properties to gasoline and thus it has the potential to be used as a substitute for gasoline in currently running engines. Clostridia are recognized as natural and good butanol producers and are employed in the industrial-scale production of solvents. Due to their complex metabolic characteristics and to the difficulty of performing genetic manipulations, in recent years the Clostridia butanol pathway was expressed in other microorganisms such as Escherichia coli and Saccharomyces cerevisiae, but in yeast the obtained results were not so promising. An alternative way for producing fusel alcohol is to exploit the degradation pathway of aminoacids released from protein hydrolysis, where proteins derive from exhausted microbial biomasses at the end of the fermentation processes. Results: It is known that wine yeasts can, at the end of the fermentation process, accumulate fusel alcohols, and butanol is among them. Despite it was quite obvious to correlate said production with aminoacid degradation, a putative native pathway was never proposed. Starting from literature data and combining information about different organisms, here we demonstrate how glycine can be the substrate for butanol and isobutanol production, individuating at least one gene encoding for the necessary activities leading to butanol accumulation. -
A Metallo-Β-Lactamase Enzyme for Internal Detoxification of The
www.nature.com/scientificreports OPEN A metallo‑β‑lactamase enzyme for internal detoxifcation of the antibiotic thienamycin Seydina M. Diene1,3, Lucile Pinault2,3, Sophie Alexandra Baron2,3, Saïd Azza1,3, Nicholas Armstrong2,3, Linda Hadjadj1,3, Eric Chabrière1,3, Jean‑Marc Rolain1,3, Pierre Pontarotti1,3,4 & Didier Raoult1,3* Thienamycin, the frst representative of carbapenem antibiotics was discovered in the mid‑1970s from soil microorganism, Streptomyces cattleya, during the race to discover inhibitors of bacterial peptidoglycan synthesis. Chemically modifed into imipenem (N‑formimidoyl thienamycin), now one of the most clinically important antibiotics, thienamycin is encoded by a thienamycin gene cluster composed of 22 genes (thnA to thnV) from S. cattleya NRRL 8057 genome. Interestingly, the role of all thn‑genes has been experimentally demonstrated in the thienamycin biosynthesis, except thnS, despite its annotation as putative β‑lactamase. Here, we expressed thnS gene and investigated its activities against various substrates. Our analyses revealed that ThnS belonged to the superfamily of metallo‑β‑lactamase fold proteins. Compared to known β‑lactamases such as OXA‑48 and NDM‑1, ThnS exhibited a lower afnity and less efciency toward penicillin G and cefotaxime, while imipenem is more actively hydrolysed. Moreover, like most MBL fold enzymes, additional enzymatic activities of ThnS were detected such as hydrolysis of ascorbic acid, single strand DNA, and ribosomal RNA. ThnS appears as a MBL enzyme with multiple activities including a specialised β‑lactamase activity toward imipenem. Thus, like toxin/antitoxin systems, the role of thnS gene within the thienamycin gene cluster appears as an antidote against the produced thienamycin. -
The Kinetics of Intramolecular Distribution of N in Uric Acid After
The Kinetics of Intramolecular Distribution of 15N in Uric Acid after Administration of [15N]Glycine A REAPPRAISAL OF THE SIGNIFICANCE OF PREFERENTIAL LABELING OF N-(3 + 9) OF URIC ACID IN PRIMARY GOUT Oded Sperling, … , James B. Wyngaarden, C. Frank Starmer J Clin Invest. 1973;52(10):2468-2485. https://doi.org/10.1172/JCI107438. Research Article The concept of an abnormality of glutamine metabolism in primary gout was first proposed on the basis of isotope data: when [15N]glycine was administered to gouty subjects, there was disproportionately great enrichment of N-(3 + 9) of uric acid, which derive from the amide-N of glutamine. An unduly high concentration of 15N in glutamine was postulated, and attributed to a hypothetical defect in catabolism of glutamine. Excess glutamine was proposed as the driving force of uric acid overproduction. We have reexamined this proposition in four gouty subjects: one mild overproducer of uric acid with “idiopathic gout,” one marked overproducer with high-grade but “partial” hypoxanthine-guanine phosphoribosyl-transferase deficiency, and two extraordinary overproducers with superactive phosphoribosylpyrophosphate synthetases. In the last three, the driving force of excessive purine biosynthesis is a known surplus of α-5-phosphoribosyl-1-pyrophosphate. Disproportionately high labeling of N-(3 + 9) was present in all four gouty subjects, most marked in the most flamboyant overproducers. The precursor glucine pool was sampled by periodic administration of benzoic acid and isolation of urinary hippuric acid. Similarly, the precursor glutamine pool was sampled by periodic administration of phenylacetic acid and isolation of the amide-N of urinary phenylacetylglutamine. The time course of 15N enrichment of hippurate differed from that of the amide-N of glutamine. -
Asn336 Is Involved in the Substrate Affinity of Glycine Oxidase From
Electronic Journal of Biotechnology 22 (2016) 26–30 Contents lists available at ScienceDirect Electronic Journal of Biotechnology Research article Asn336 is involved in the substrate affinity of glycine oxidase from Bacillus cereus Gaobing Wu a, Tao Zhan b,YimingGuob, Ashok Kumar b, Ziduo Liu b,⁎ a State Key Laboratory of Agricultural Microbiology, College of Plant Science and Technology, Huazhong Agricultural University, Wuhan 430070, China b State Key Laboratory of Agricultural Microbiology, College of Life Science and Technology, Huazhong Agricultural University, Wuhan 430070, China article info abstract Article history: Background: Glycine oxidase (GO), a type of D-amino acid oxidase, is of biotechnological interest for its potential Received 5 October 2015 in several fields. In our previous study, we have characterized a new glycine oxidase (BceGO) from Bacillus cereus Accepted 13 May 2016 HYC-7. Here, a variant of N336K with increased the affinity against all the tested substrate was obtained by Available online 05 June 2016 screening a random mutant library of BceGO. It is observed that the residue N336 is invariable between its homogeneous enzymes. This work was aimed to explore the role of the residue N336 in glycine oxidase by Keywords: site-directed mutagenesis, kinetic assay, structure modeling and substrate docking. Bacillus cereus Results: The results showed that the affinity of N336H, N336K and N336R increased gradually toward all the Error-prone PCR Glycine oxidase substrates, with increase in positive charge on side chain, while N336A and N336G have not shown a little Site-directed mutagenesis significant effect on substrate affinity. The structure modeling studies indicated that the residue Asn336 is Substrate affinity located in a random coil between β-18 and α-10. -
Classification I Classification II Subsystem Functional Role
Classification I Classification II Subsystem Functional Role Occurrence Amino Acids and Derivatives Alanine, serine, and glycine Alanine Biosynthesis Cysteine desulfurase (EC 4.4.1.-) 2 Amino Acids and Derivatives Alanine, serine, and glycine Glycine cleavage system Glycine dehydrogenase [decarboxylating] (glycine cleavage system P protein) (EC 1.4.4.2) 4 Amino Acids and Derivatives Alanine, serine, and glycine Glycine cleavage system Dihydrolipoamide dehydrogenase (EC 1.8.1.4) 3 Amino Acids and Derivatives Alanine, serine, and glycine Glycine cleavage system Aminomethyltransferase (glycine cleavage system T protein) (EC:2.1.2.10) 1 Amino Acids and Derivatives Alanine, serine, and glycine Glycine synthesis Serine hydroxymethyltransferase (EC 2.1.2.1) 2 Amino Acids and Derivatives Alanine, serine, and glycine Glycine synthesis Low- specificity L-threonine aldolase (EC 4.1.2.5) 2 Amino Acids and Derivatives Alanine, serine, and glycine Serine Biosynthesis Phosphoserine aminotransferase (EC 2.6.1.52) 1 Amino Acids and Derivatives Alanine, serine, and glycine Serine Biosynthesis Phosphoserine phosphatase (EC 3.1.3.3) 1 Amino Acids and Derivatives Alanine, serine, and glycine Serine Biosynthesis Serine hydroxymethyltransferase (EC 2.1.2.1) 2 Amino Acids and Derivatives Arginine; urea cycle, polyamines Arginine Putrescine and 4- aminobutyrate degradation Urease accessory protein UreF 3 Amino Acids and Derivatives Arginine; urea cycle, polyamines Arginine Putrescine and 4- aminobutyrate degradation Agmatine deiminase (EC 3.5.3.12) 1 Amino Acids -
Deamination Subhadipa 2020 Fate of Amino Acid
Subhadipa 2020 Deamination Subhadipa 2020 Fate of Amino acid • Deamination is the removal of an α amino group from a molecule. Amino group is converted into ammonia while the amino acid itself converts into its corresponding keto acid. • Enzymes that catalyse this reaction are called deaminases. • In the human body, deamination takes place primarily in the liver, however it is also deaminated in the kidney. Oxidative deamination Subhadipa 2020 Oxidative deamination is stereospecific and is catalyzed by L- or D-amino acid oxidase. The initial step is removal of two hydrogen atoms by the flavin coenzyme, with formation of an unstable α-amino acid intermediate. This intermediate undergoes decomposition by addition of water and forms the ammonium ion and the corresponding α-keto acid. Presence of O2 is essential. By the help of L-amino acid oxidase (LAAO) • Enzyme present in mitochondria, peroxisomes and ER of mammalian kidneys and liver only. • It contains FMN/FAD as the prosthetic group. • It can’t act on glycine and L-isomers basic amino acids. H2O Subhadipa 2020 By the help of D-amino acid oxidase (DAO) It occurs in peroxisomes of mammalian liver and kidneys. It can’t act on D-isomers of glutamic acid, asparagine, dicarboxylic acid and basic amino acids. It contains FAD as prosthetic group. Subhadipa 2010 By the help of Glutamate dehydrogenase • It is present in all tissue. • It needs NAD or NADP as coenzyme. By the help of Subhadipa 2020 Glycine oxidase • FAD as a prosthetic group. • Act on glycine. Subhadipa 2020 Non-oxidative deamination Molecular O2 is non essential. -
Expression of an Evolved Engineered Variant of a Bacterial Glycine
Journal of Biotechnology 184 (2014) 201–208 Contents lists available at ScienceDirect Journal of Biotechnology j ournal homepage: www.elsevier.com/locate/jbiotec Expression of an evolved engineered variant of a bacterial glycine oxidase leads to glyphosate resistance in alfalfa a,∗ a b,c a b,c a A. Nicolia , N. Ferradini , G. Molla , E. Biagetti , L. Pollegioni , F. Veronesi , a D. Rosellini a Dipartimento di Scienze Agrarie, Alimentari e Ambientali, University of Perugia, Borgo XX Giugno, 74, 06121 Perugia, Italy b Dipartimento di Biotecnologie e Scienze della Vita, Università degli studi dell’Insubria, via J.H. Dunant 3, 21100 Varese, Italy c Centro Interuniversitario di Ricerca in Biotecnologie Proteiche The Protein Factory, Politecnico di Milano, ICRM CNR Milano and Università degli studi dell’Insubria, via Mancinelli 7, 20131 Milano, Italy a r t i c l e i n f o a b s t r a c t Article history: The main strategy for resistance to the herbicide glyphosate in plants is the overexpression of an herbicide Received 16 January 2014 insensitive, bacterial 5-enolpyruvylshikimate-3-phosphate synthase (EPSPS). A glyphosate resistance Received in revised form 14 May 2014 strategy based on the ability to degrade the herbicide can be useful to reduce glyphosate phytotox- Accepted 16 May 2014 icity to the crops. Here we present the characterization of glyphosate resistance in transgenic alfalfa Available online 4 June 2014 (Medicago sativa L.) expressing a plant-optimized variant of glycine oxidase (GO) from Bacillus subtilis, evolved in vitro by a protein engineering approach to efficiently degrade glyphosate. Two constructs Keywords: TP+ TP− were used, one with (GO ) and one without (GO ) the pea rbcS plastid transit peptide. -
Springer Handbook of Enzymes
Dietmar Schomburg Ida Schomburg (Eds.) Springer Handbook of Enzymes Alphabetical Name Index 1 23 © Springer-Verlag Berlin Heidelberg New York 2010 This work is subject to copyright. All rights reserved, whether in whole or part of the material con- cerned, specifically the right of translation, printing and reprinting, reproduction and storage in data- bases. The publisher cannot assume any legal responsibility for given data. Commercial distribution is only permitted with the publishers written consent. Springer Handbook of Enzymes, Vols. 1–39 + Supplements 1–7, Name Index 2.4.1.60 abequosyltransferase, Vol. 31, p. 468 2.7.1.157 N-acetylgalactosamine kinase, Vol. S2, p. 268 4.2.3.18 abietadiene synthase, Vol. S7,p.276 3.1.6.12 N-acetylgalactosamine-4-sulfatase, Vol. 11, p. 300 1.14.13.93 (+)-abscisic acid 8’-hydroxylase, Vol. S1, p. 602 3.1.6.4 N-acetylgalactosamine-6-sulfatase, Vol. 11, p. 267 1.2.3.14 abscisic-aldehyde oxidase, Vol. S1, p. 176 3.2.1.49 a-N-acetylgalactosaminidase, Vol. 13,p.10 1.2.1.10 acetaldehyde dehydrogenase (acetylating), Vol. 20, 3.2.1.53 b-N-acetylgalactosaminidase, Vol. 13,p.91 p. 115 2.4.99.3 a-N-acetylgalactosaminide a-2,6-sialyltransferase, 3.5.1.63 4-acetamidobutyrate deacetylase, Vol. 14,p.528 Vol. 33,p.335 3.5.1.51 4-acetamidobutyryl-CoA deacetylase, Vol. 14, 2.4.1.147 acetylgalactosaminyl-O-glycosyl-glycoprotein b- p. 482 1,3-N-acetylglucosaminyltransferase, Vol. 32, 3.5.1.29 2-(acetamidomethylene)succinate hydrolase, p. 287 Vol.