Smart Hydrogels Based Platforms for Investigation of Biochemical Reactions
Total Page:16
File Type:pdf, Size:1020Kb
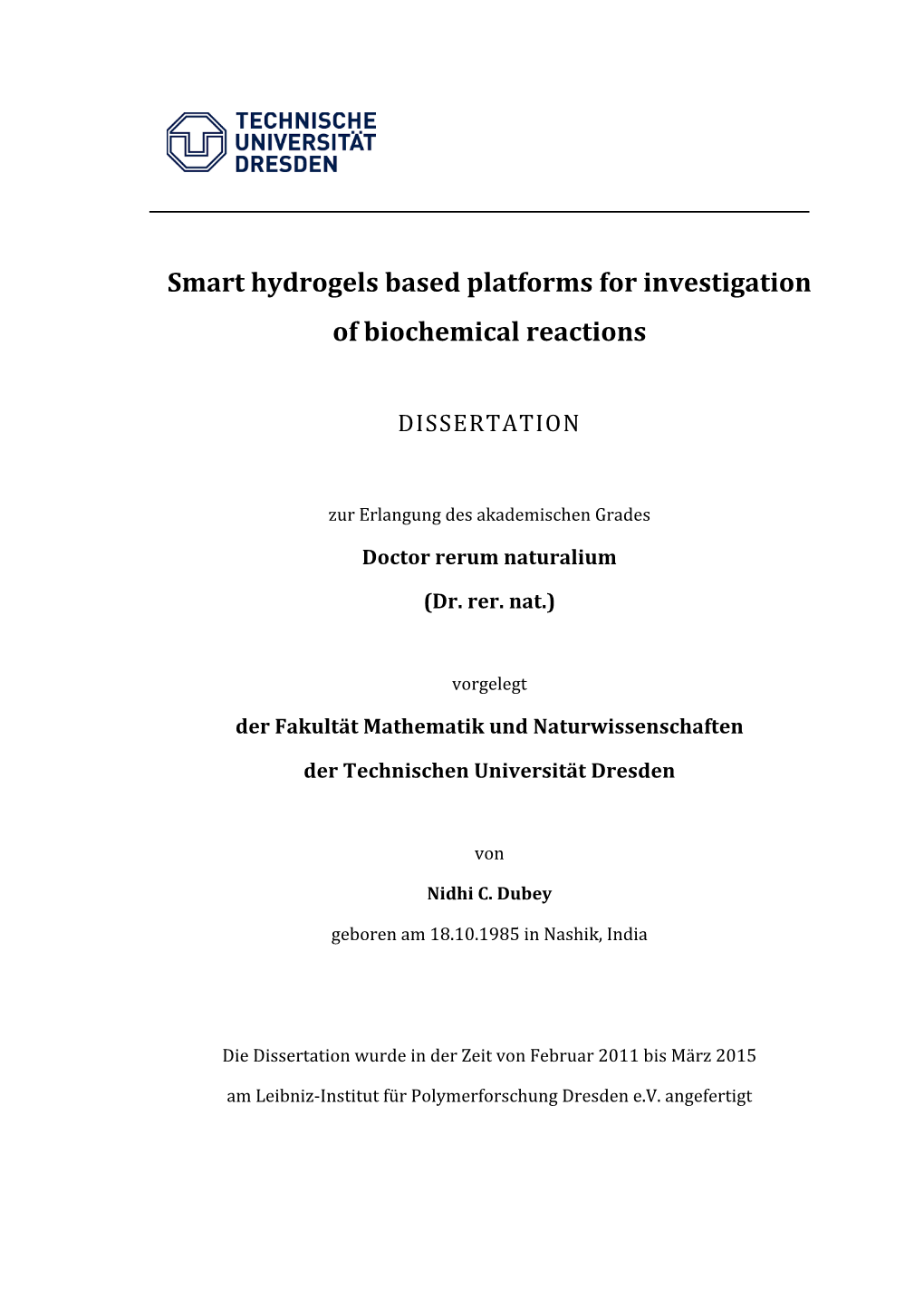
Load more
Recommended publications
-
Preparation and Characterization of Thermoresponsive Poly(N-Isopropylacrylamide) for Cell Culture Applications
polymers Review Preparation and Characterization of Thermoresponsive Poly(N-Isopropylacrylamide) for Cell Culture Applications Lei Yang 1,* , Xiaoguang Fan 2,*, Jing Zhang 1 and Jia Ju 1 1 College of Chemistry, Chemical Engineering and Environmental Engineering, Liaoning Shihua University, Fushun 113001, China; [email protected] (J.Z.); [email protected] (J.J.) 2 College of Engineering, Shenyang Agricultural University, Shenyang 110866, China * Correspondence: [email protected] (L.Y.); [email protected] (X.F.); Tel.: +86-024-5686-1705 (L.Y.); +86-024-8848-7119 (X.F.) Received: 7 November 2019; Accepted: 17 January 2020; Published: 9 February 2020 Abstract: Poly(N-isopropylacrylamide) (PNIPAAm) is a typical thermoresponsive polymer used widely and studied deeply in smart materials, which is attractive and valuable owing to its reversible and remote “on–off” behavior adjusted by temperature variation. PNIPAAm usually exhibits opposite solubility or wettability across lower critical solution temperature (LCST), and it is readily functionalized making it available in extensive applications. Cell culture is one of the most prospective and representative applications. Active attachment and spontaneous detachment of targeted cells are easily tunable by surface wettability changes and volume phase transitions of PNIPAAm modified substrates with respect to ambient temperature. The thermoresponsive culture platforms and matching thermal-liftoff method can effectively substitute for the traditional cell harvesting ways like enzymatic hydrolysis and mechanical scraping, and will improve the stable and high quality of recovered cells. Therefore, the establishment and detection on PNIPAAm based culture systems are of particular importance. This review covers the important developments and recommendations for future work of the preparation and characterization of temperature-responsive substrates based on PNIPAAm and analogues for cell culture applications. -
The Characteristics of the Smart Polymeras Temperature Or Ph- Responsive Hydrogel
Available online at www.sciencedirect.com ScienceDirect Procedia Chemistry 19 ( 2016 ) 406 – 409 5th International Conference on Recent Advances in Materials, Minerals and Environment (RAMM) & 2nd International Postgraduate Conference on Materials, Mineral and Polymer (MAMIP), 4-6 August 2015 The Characteristics of the Smart Polymeras Temperature or pH- responsive Hydrogel B. Hilmia, Z.A. Abdul Hamida*, H. Md Akila, and B.H. Yahayab aBiomaterials Niche Group, School of Materiasl and Mineral Resources Engineering, Universiti Sains Malaysia, 14300, Nibong Tebal, Pulau Pinang, Malaysia bRegenerative Medicine Cluster, Advanced Medical and Dental Institute, Bertam,13200 Kepala Batas, Pulau Pinang Malaysia Abstract Hydrogels have unique swelling behaviour and three-dimensional structure and can be applied in biomedical and tissue engineering fields. Hydrogels also can be prepared by several methods. They are called as smart hydrogels as they able to undergo transitional changes in response to environmental stimuli. One of the stimuli is temperature. The temperature can create changes to the smart polymer as they have a very sensitive balance between the hydrophobic and the hydrophilic groups in their structure. Some hydrogels exhibit a separation from solution and solidification above a certain temperature. This threshold is known as the lower critical solution temperature (LCST). The other stimulus is pH which also can give effect to hydrogel in order to be further applied as drug delivery agents. The main feature of this kind of smart polymer is an ability to receive or release protons which responding to the pH changes. These polymers are polyelectrolyte where containing acid groups or basic groups. Temperature and pH responsive hydrogel is essential and currently investigated in many various applications specifically in drug delivery system due to their unique responsive characteristics. -
Designing Smart Biomaterials for Tissue Engineering
International Journal of Molecular Sciences Opinion Designing Smart Biomaterials for Tissue Engineering Ferdous Khan 1,*,† and Masaru Tanaka 1,2,* 1 Soft-Materials Chemistry, Institute for Materials Chemistry and Engineering, Kyushu University, 744 Motooka Nishi-ku, Fukuoka 819-0395, Japan 2 Frontier Center for Organic Materials, Yamagata University, 4-3-16 Jonan, Yonezawa, Yamagata 992-8510, Japan * Correspondence: [email protected] (F.K.); [email protected] or [email protected] (M.T.) † Current address: ECOSE-Biopolymer, Knauf Insulation Limited, P.O. Box 10, Stafford Road, ST. HELENS WA10 3NS, UK. Received: 1 November 2017; Accepted: 1 December 2017; Published: 21 December 2017 Abstract: The engineering of human tissues to cure diseases is an interdisciplinary and a very attractive field of research both in academia and the biotechnology industrial sector. Three-dimensional (3D) biomaterial scaffolds can play a critical role in the development of new tissue morphogenesis via interacting with human cells. Although simple polymeric biomaterials can provide mechanical and physical properties required for tissue development, insufficient biomimetic property and lack of interactions with human progenitor cells remain problematic for the promotion of functional tissue formation. Therefore, the developments of advanced functional biomaterials that respond to stimulus could be the next choice to generate smart 3D biomimetic scaffolds, actively interacting with human stem cells and progenitors along with structural integrity to form functional tissue within a short period. To date, smart biomaterials are designed to interact with biological systems for a wide range of biomedical applications, from the delivery of bioactive molecules and cell adhesion mediators to cellular functioning for the engineering of functional tissues to treat diseases. -
Smart Polymers in Micro and Nano Sensory Devices
chemosensors Review Smart Polymers in Micro and Nano Sensory Devices José Antonio Reglero Ruiz * ID , Ana María Sanjuán, Saúl Vallejos, Félix Clemente García and José Miguel García ID Departamento de Química Orgánica, Facultad de Ciencias, Universidad de Burgos, Plaza Misael Bañuelos s/n, 09001 Burgos, Spain; [email protected] (A.M.S.); [email protected] (S.V.); [email protected] (F.C.G.); [email protected] (J.M.G.) * Correspondence: [email protected]; Tel.: +34-947-258-085 Received: 25 February 2018; Accepted: 19 March 2018; Published: 21 March 2018 Abstract: The present review presents the most recent developments concerning the application of sensory polymers in the detection and quantification of different target species. We will firstly describe the main polymers that are being employed as sensory polymers, including, for example, conducting or acrylate-based polymers. In the second part of the review, we will briefly describe the different mechanisms of detection and the target species, such as metal cations and anions, explosives, and biological and biomedical substances. To conclude, we will describe the advancements in recent years concerning the fabrication of micro and nano sensory devices based on smart polymers, with a bibliographic revision of the research work published between 2005 and today, with special emphasis on research work presented since 2010. A final section exposing the perspectives and challenges of this interesting research line will end the present review article. Keywords: sensors; smart polymers; detection; sensory devices 1. Introduction Sensory or smart polymers present the ability to respond, reversibly or irreversibly, to different stimuli. Different sources can cause these responses: Temperature [1], electromagnetic pulses [2], biological molecules [3] or Ph media [4]. -
Photoresponsive Polymer–Enzyme Switches
Photoresponsive polymer–enzyme switches Tsuyoshi Shimoboji*, Edmund Larenas†, Tim Fowler†, Samarth Kulkarni*, Allan S. Hoffman*‡, and Patrick S. Stayton*‡ *Department of Bioengineering, University of Washington, Seattle, WA 98195; and †Genencor International, Inc., Palo Alto, CA 94304 Edited by James A. Wells, Sunesis Pharmaceuticals, South San Francisco, CA, and approved October 30, 2002 (received for review July 18, 2002) The ability to photoregulate enzyme activities could provide im- Materials and Methods portant new opportunities for development of diagnostic assays, Materials. 4-hydroxyazobenzene and 4-aminoazobenzene sequential bioprocessing, and lab assays in both traditional and (Aldrich) were recrystallized from ethanol and water, and dried microfluidic formats. We show here that the photoinduced in vacuo. N,N-dimethyl acrylamide (DMA) (Fluka), diethyl changes in the size and hydration of a ‘‘smart’’ polymer chain coil ether, acryloylchloride, triethylamine, 2-mercaptoethanol (Al- can be used to regulate substrate access and enzyme activity when drich) were purified by distillation under reduced pressure. conjugated to the enzyme at a specific point just outside the active 2,2Ј-azobisbutyronitrile (J. T. Baker, Phillipsburg, NJ) was re- site. The photoresponsive polymers thus serve jointly as antennae crystallized from methanol. Ethanol, dimethylformamide, tet- and actuators that reversibly respond to distinct optical signals to rahydrofuran, methylene chloride, potassium ter-butoxide, switch the polymer–enzyme conjugates on and off, and work 4-phenylazomaleinanil, divinylsulfone, 4-phenylazomaleinanil when the conjugate is free in solution or when immobilized on and EDTA (Aldrich) were used as received. Restriction enzymes magnetic beads. XbaI and BglII were purchased from New England Biolabs. QIA Prep Spin Plasmid kit (Qiagen, Valencia, CA) and QuikChange smart polymer ͉ photoswitch ͉ biotechnology ͉ bioMEMs ͉ bioconjugates Site-Directed Mutagenesis kit (Stratagene) were used as re- ceived. -
Design and Applications of Responsive Polymers In
Design and Applications of Site-specific smart polymer-protein Responsive Polymers in conjugates: We have extended this technology to site-specific protein-polymer Diagnostics, Separations, conjugates, where the conjugation site is Bioprocesses, and Drug Delivery near the protein’s active site. To do this we use site-specific mutagenesis to form mutant Allan S. Hoffman, Patrick S. Stayton proteins with cysteine -SH groups near the plus many students, postdocs and active site, to which we conjugate our smart collaborators, polymer. This permits us to block and Bioengineering Department, University unblock the active, recognition site of the of Washington, Box 355061, Seattle, protein by different stimuli, and sometimes to do this without phase separation of the WA 98195 conjugate. [email protected] Random conjugates of smart polymers Dual-responsive smart copolymers: We and proteins: We have been designing and have synthesized polymers and copolymers synthesizing a wide variety of stimuli- with dual responsivities to combinations of responsive polymers (which are sometimes temperature, pH and light (visible-UV) called "smart" polymers) for many different stimuli. The polymer structures may be applications in biotechnology and medicine. random, block or graft copolymers. Most We have conjugated or complexed the recently we have used living free radical responsive polymers to (a) proteins such as polymerization techniques such as RAFT to streptavidin, antibodies and enzymes, (b) form block copolymers with two different nucleic acids and (c) a variety of drugs. sensitivity blocks. We have demonstrated Random conjugation of the smart polymer to control of an enzyme bioprocess using both a protein is usually carried out by reaction of light- and temperature-sensitive copolymer- a terminal or pendant group of the polymer enzyme conjugates. -
Electroactive Smart Polymers for Biomedical Applications
materials Review Electroactive Smart Polymers for Biomedical Applications Humberto Palza 1,2,*, Paula Andrea Zapata 3 and Carolina Angulo-Pineda 1 1 Departamento de Ingeniería Química, Biotecnología y Materiales, Facultad de Ciencias Físicas y Matemáticas, Universidad de Chile, 8370456 Santiago, Chile; [email protected] 2 Millenium Nuclei in Soft Smart Mechanical Metamaterials, Universidad de Chile, 8370456 Santiago, Chile 3 Grupo de Polímeros, Facultad de Química y Biología, Universidad de Santiago de Chile, 8350709 Santiago, Chile; [email protected] * Correspondence: [email protected]; Tel.: +56-229-784-085 Received: 6 December 2018; Accepted: 9 January 2019; Published: 16 January 2019 Abstract: The flexibility in polymer properties has allowed the development of a broad range of materials with electroactivity, such as intrinsically conductive conjugated polymers, percolated conductive composites, and ionic conductive hydrogels. These smart electroactive polymers can be designed to respond rationally under an electric stimulus, triggering outstanding properties suitable for biomedical applications. This review presents a general overview of the potential applications of these electroactive smart polymers in the field of tissue engineering and biomaterials. In particular, details about the ability of these electroactive polymers to: (1) stimulate cells in the context of tissue engineering by providing electrical current; (2) mimic muscles by converting electric energy into mechanical energy through an electromechanical response; -
The Synthesis and Development of Novel, Easily Processable Poly (N-Isopropylacrylamide)-Based Hyrdogels
The synthesis and development of novel, easily processable poly (n-isopropylacrylamide)-based hyrdogels. BOYES, Victoria L. Available from Sheffield Hallam University Research Archive (SHURA) at: http://shura.shu.ac.uk/19381/ This document is the author deposited version. You are advised to consult the publisher's version if you wish to cite from it. Published version BOYES, Victoria L. (2012). The synthesis and development of novel, easily processable poly (n-isopropylacrylamide)-based hyrdogels. Doctoral, Sheffield Hallam University (United Kingdom).. Copyright and re-use policy See http://shura.shu.ac.uk/information.html Sheffield Hallam University Research Archive http://shura.shu.ac.uk 1 L.ceimiiiy ana inTQrmation Services I I Adsetts Centre, City Campus I | Sheffield S 1 1VVD _ _ | 102 019 751 X REFERENCE ProQuest Number: 10694262 All rights reserved INFORMATION TO ALL USERS The quality of this reproduction is dependent upon the quality of the copy submitted. In the unlikely event that the author did not send a complete manuscript and there are missing pages, these will be noted. Also, if material had to be removed, a note will indicate the deletion. uest ProQuest 10694262 Published by ProQuest LLC(2017). Copyright of the Dissertation is held by the Author. All rights reserved. This work is protected against unauthorized copying under Title 17, United States Code Microform Edition © ProQuest LLC. ProQuest LLC. 789 East Eisenhower Parkway P.O. Box 1346 Ann Arbor, Ml 48106- 1346 The Synthesis and Development of Novel, Easily Processable Poly{ N- Isopropylacrylamide)- Based Hydrogels Victoria Boyes A thesis submitted in partial fulfilment of the requirements of Sheffield Hallam University for the degree of Doctor of Philosophy September 2012 Collaborating Organisation: Smith & Nephew Extruded Films Declaration The work described in this thesis was carried out by the author in the Materials and Engineering Research Institute at Sheffield Hallam University, between October 2008 and August 2012. -
Development of Dual-Sensitive Smart Polymers by Grafting Chitosan with Poly (N-Isopropylacrylamide): an Overview
http://dx.doi.org/10.1590/0104-1428.1744 Development of dual-sensitive smart polymers by grafting O chitosan with poly (N-isopropylacrylamide): an overview V 1 1* 1 Nívia do Nascimento Marques , Ana Maria da Silva Maia and Rosangela de Carvalho Balaban E 1Laboratório de Pesquisa em Petróleo, Instituto de Química, Universidade Federal do Rio Grande do Norte – UFRN, Natal, RN, Brasil R *[email protected] V Abstract A great deal of research on polymers over the past two decades has been focused on the development of stimuli-responsive I polymers to obtain materials able to respond to specific surroundings. In this paper, an overview is presented of the concepts, behavior and applicability of these “smart polymers”. Polymers that are temperature- or pH-sensitive are E discussed in detail, including the response mechanisms and types of macromolecules, because they are easy to handle and have a wide range of applications. Finally, the combination of pH and temperature responsive properties by means of graft copolymerization of chitosan with poly (N-isopropylacrylamide) (PNIPAM) was chosen to represent some W synthetic routes and properties of dual-sensitive polymeric systems developed currently. Keywords: smart polymer, thermosensitive, pH-responsive, N-isopropylacrylamide, chitosan. 1. Introduction Recently, scientists all over the world have been material[41,42]. A quite common approach involves preparing attempting to synthesize polymers capable of mimic the polymers sensible to both temperature and pH stimuli[43-55], stimuli-responsive property present in common biopolymers since they are typical variables parameters in biological and of living organisms, in order to reach scientific and industrial chemical systems, and they can also be easily controlled in applications. -
“Smart” Holograms – a Novel Diagnostics Platform
“Smart” Holograms – A Novel Diagnostics Platform. S. Kabilan*,A.J.Marshall,A.Horgan,C.D.Creasey,S.J.Kew,K.E.S.Dean,S.F.Terrell, L. J. Affleck. Smart Holograms Ltd., 291 Cambridge Science Park, Milton Road, Cambridge CB4 0WF, United Kingdom, *[email protected] ABSTRACT laser (532 nm) [2]. After a conventional photographic development step, illumination of the grating under white “Smart” holograms are novel sensor systems which utilise light recreates the monochromatic image of the plane diffraction as the transduction method for the detection of mirror used in its construction with the constructive physical stimuli or (bio)chemical analytes. Interaction of interference at each fringe plane resulting in a characteristic these sensors with a specific analyte or stimulus changes spectral peak with a wavelength approximately governed by the colour, image or brightness of the hologram and these Bragg’s Law: changes can be visualised directly by the user or quantified mO = 2ndsinT using a simple colour reader. Sensor holograms are inexpensive, robust, label-free, format flexible systems where m is the diffraction order, O is the wavelength of which can be engineered to be sensitive to a wide range of light in vacuo, n is the average refractive index of the analytes. system, d is the spacing of the diffracting plane and T is the glancing angle between the incident light propagation Keywords: Hologram, Sensor, Diffraction, Glucose direction and the diffracting planes. Any physical or (bio)chemical mechanism that changes spacing of the 1 INTRODUCTION fringes (d) or the average refractive index (n) will generate observable changes in the wavelength (colour) of the Sensor holograms utilise the principles of volume reflection hologram. -
Poly(N-Isopropylacrylamide) and Copolymers: a Review on Recent Progresses in Biomedical Applications
Review Poly(N-isopropylacrylamide) and Copolymers: A Review on Recent Progresses in Biomedical Applications Sonia Lanzalaco 1 and Elaine Armelin 2,3,* 1 Industrial and Digital Innovation Department (DIID), Chemical Engineering, University of Palermo, Viale delle Scienze, Ed. 8, 90128 Palermo, Italy; [email protected] 2 Departament d’Enginyeria Química, EEBE, Universitat Politècnica de Catalunya, C/d’Eduard Maristany, 10-14, Building I, E-08019 Barcelona, Spain 3 Barcelona Research Center in Multiscale Science and Engineering, Universitat Politècnica de Catalunya, Campus Diagonal Besòs (EEBE), C/d’Eduard Maristany 10-14, Edifici IS, 08019 Barcelona, Spain * Correspondence: [email protected]; Tel.: +34-934-054-4447 Received: 15 August 2017; Accepted: 3 October 2017; Published: 4 October 2017 Abstract: The innate ability of poly(N-isopropylacrylamide) (PNIPAAm) thermo-responsive hydrogel to copolymerize and to graft synthetic polymers and biomolecules, in conjunction with the highly controlled methods of radical polymerization which are now available, have expedited the widespread number of papers published in the last decade—especially in the biomedical field. Therefore, PNIPAAm-based hydrogels are extensively investigated for applications on the controlled delivery of active molecules, in self-healing materials, tissue engineering, regenerative medicine, or in the smart encapsulation of cells. The most promising polymers for biodegradability enhancement of PNIPAAm hydrogels are probably poly(ethylene glycol) (PEG) and/or poly(ε- caprolactone) (PCL), whereas the biocompatibility is mostly achieved with biopolymers. Ultimately, advances in three-dimensional bioprinting technology would contribute to the design of new devices and medical tools with thermal stimuli response needs, fabricated with PNIPAAm hydrogels. Keywords: poly(N-isopropylacrylamide); thermo-responsive polymer; copolymers; biocompatibility; biodegradability; 4D-printing 1. -
Stimuli-Responsive Drug Release from Smart Polymers
Journal of Functional Biomaterials Review Stimuli-Responsive Drug Release from Smart Polymers Carlos M. Wells * , Michael Harris, Landon Choi, Vishnu Priya Murali, Fernanda Delbuque Guerra and J. Amber Jennings Department of Biomedical Engineering, The University of Memphis, Memphis, TN 38152, USA * Correspondence: [email protected]; Tel.: +1-1901-289-9827 Received: 28 May 2019; Accepted: 26 July 2019; Published: 31 July 2019 Abstract: Over the past 10 years, stimuli-responsive polymeric biomaterials have emerged as effective systems for the delivery of therapeutics. Persistent with ongoing efforts to minimize adverse effects, stimuli-responsive biomaterials are designed to release in response to either chemical, physical, or biological triggers. The stimuli-responsiveness of smart biomaterials may improve spatiotemporal specificity of release. The material design may be used to tailor smart polymers to release a drug when particular stimuli are present. Smart biomaterials may use internal or external stimuli as triggering mechanisms. Internal stimuli-responsive smart biomaterials include those that respond to specific enzymes or changes in microenvironment pH; external stimuli can consist of electromagnetic, light, or acoustic energy; with some smart biomaterials responding to multiple stimuli. This review looks at current and evolving stimuli-responsive polymeric biomaterials in their proposed applications. Keywords: stimuli-responsiveness; drug release; drug delivery; thermo-responsive materials; enzyme-responsive materials; pH-responsive materials; shape-memory materials 1. Introduction Researchers throughout different disciplines continue to explore improved and safer ways to locally deliver drugs to specific sites of action, attempting to increase specificity and efficacy. Over the years, numerous investigations on biomaterials have seen successes in the development of controlled delivery systems [1,2].